
книги / Nanotechnology Read and Discuss
..pdf
Mechanical properties of small material volumes
The strength of submicron-sized metals and ceramics is considerably higher than their bulk forms. The fracture strength of brittle glasses and ceramics is strongly size dependent, due to the decreasing chance for the material to contain large
flaws as its size reduces. Thus, glass and carbon fibers are orders of magnitude stronger than their bulk counterparts, and they are highly flexible so they are effective strengtheners and stiffeners in plastic-based composites. Compared to fibers, nanotubes and nanowires are even stronger and more
flexible, although accurate evaluation of fracture stresses in these materials is difficult. Carbon nanotubes are known to have high fracture strains of 10–30% and excep-
tionally high Young’s moduli of the order of TPa. For metals sub-millimeter-sized whiskers may exhibit sharp yield points even when bulk-sized samples of the same material do not (e.g. Cu). The occurrence of the sharp yield point in this case is due to the necessity to generate dislocations from the perfect lattice, and the upper yield point (fig. 6) may approach the theoretical yield strength of E/10.
Similar observations were also made in compression experiments on micronto submicron-sized metallic crystals using a flat-end diamond punch (fig. 7) in a nanoindenter.
51

Fig. 8 shows that as the column size falls into the submicron regime in the case of Ni3Al, the
elastic-to-plastic transition becomes very abrupt. The GPa level yield stress here is an order of magnitude higher
than the yield stress of the bulk sample, which does not exhibit a sharp yield phenomenon. While the yield stress of a submicron-sized crystal may be as high as several GPa, its thermal stability may not be great. Under a GPa-level elastic stress, although the perfect crystal environment is momentarily maintained, the atomic planes sandwiching a potential slip plane may be sheared very close to the instability point (fig. 8).
Fig. 8. Stress–strain curves of Ni3Al microcolumns (a); SEM image of a microcolumn produced by focused-ion milling (b); post-compression
image of a column (c) (Uchic et al., 2005, courtesy of the American Association for the Advancement of Science)
Such a small margin to instability can easily be overcome by thermal agitation. Fig. 9, а shows results from a nanoindentation experiment
52
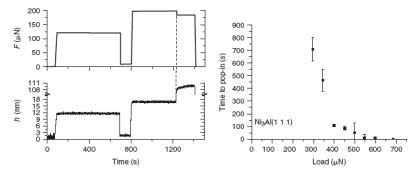
on a well-annealed aluminum crystal at room temperature. The stressed region underneath the indenter was of the order of 100 nm across and the average initial dislocation spacing in the annealed state (>>10µm) was such that no dislocation could be expected to be present inside the stressed volume. In the first loading cycle, no plasticity was created during the 600 s holding at 120µN, and the stressed volume recovered elastically upon load removal. However, during the next cycle at 200µN, the stressed volume survived elastically only for about 450 s, after which plasticity occurred followed by creep. The delay of 450 s in this case is thought to be due to the occurrence of a big enough lattice wave to trigger instability at some point within the stressed volume. Such a waiting time in general increases as the holding load decreases, as shown in fig. 9, b in the case of Ni3Al.
a |
b |
Fig. 9. Load ( F) and tip displacement (h) vs time data in
a nanoindentation experiment at room temperature on an annealed aluminum crystal using a sharp Berkovich tip (Feng and Ngan, 2001) (a); Relationship between waiting time for plasticity and holding load in Ni3Al
(Chiu and Ngan, 2001) (b)
The size and time dependence of the elastic-to-plastic transition is unique to small crystals, and is a phenomenon that must be sufficiently understood before the high-strength advantage of small crystals can be utilized in applications. In addition to the onset of plasticity, the flow resistance of submicron crystals in situations with strong strain gradients
53
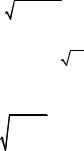
(e.g. torsion, indentation) also exhibits a remarkable strengthening effect as the material size decreases. The torsional strength of metallic whiskers, as well as the nanoindentation hardness on crystalline specimens, often exhibit a significant rise as the material size decreases (fig. 10, a). Both deformation situations feature strong plastic strain gradients which have to be accommodated by geometrically necessary dislocations (Section 6.6.2.4). In the Nix–Gao model, the rise in hardness in a small indent is modeled as a work-hardening effect due to the geometrically necessary dislocations (GNDs) in the plastic zone. As shown in fig. 10, b, the GNDs produce steps of average spacing s = ba / h on the specimen surface, where b is the Burgers vector, and a and h are the radius and depth of the indent (assumed to be conical). If λ is the total line length of the GNDs, the portion stored in an annulus ring of radius r and thickness dr is dλ = 2πr (dr / s) = 2πr (h / ba) dr, and so
λ = ∫a |
h |
2πrdr = |
πha . |
|
|||
0 |
ba |
|
b |
Assuming that all GNDs reside inside a hemisphere with the same radius a as the indent, the density of the GNDs is given by
ρG = |
λ |
= |
3h |
= |
3 |
tan |
2 |
θ. |
(12.1) |
(2 / 3)πa3 |
2ba2 |
2bh |
|
The amount of work hardening is given by Taylor’s model (Section 6.6.2.1) to be
τ=αµb ρG +ρs, |
(12.2) |
where ρs is the density of the ‘statistically stored’ dislocation population. Assuming that the direct stress σ = 3τ and that the hardness H =3σ (Section 6.1.3), with equations (12.1) and (12.2), the hardness is given by
H |
= |
1 |
+ |
h * |
, |
(12.3) |
|
h |
|||||
H0 |
|
|
|
|
where H0 and h* are constants. Equation (12.3) predicts that the hardness increases as the indent size h decreases. This equation was found to
54

rather accurately describe the hardness data such as those in fig. 10, a within a certain indent depth range.
Despite the impressive strength of small crystal volumes, their plastic deformation following
the first yield point is usually very jumpy, exhibiting sporadic, discrete strain bursts. Fig. 11, a shows the stressstrain curve of an aluminum micro-column compressed according to the load schedule
shown in the inset diagram. The discrete strain bursts are seen as large discontinuities in the stress-strain graph. The post-deformation micrograph in fig. 11, b reveals many slip steps on the column’s free surface, and each of these is likely to be associated with a strain burst in fig. 11, a.
a |
b |
Fig. 10. Nanoindentation hardness at varying indent depths for MgO (Feng and Nix, 2004) (a); Geometrically necessary dislocations within the plastic zone of an indent (Nix and Gao, 1998) (b)
Small crystalline materials may thus be difficult to be formed into smooth shapes by plastic deformation. The creep deformation of small crystal volumes, even under low temperatures relative to melting, also needs special attention. The lower panel of fig. 9, a indicates that imme-
55
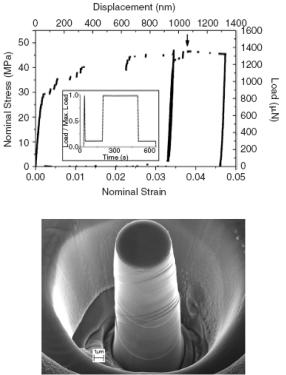
diately after the onset of plasticity at 1205 s, the indenter displacement h increased gradually with time, implying that the specimen underwent creep deformation under fixed indentation force at room temperature.
a
b
Fig. 11. Stress-strain curve of an aluminum micro-column during compression experiment by a flat-ended nanoindenter. The load schedule used is shown in the inset (a); SEM micrograph of the deformed column (b) (K.S. Ng and A.H.W. Ngan, unpublished)
That diffusion is important in the low-temperature deformation of nanocrystalline materials can be seen from the few unusual mechanical characteristics of these materials as compared with their ordinary polycrystalline counterparts. First, their grain-boundary fraction is a lot
56
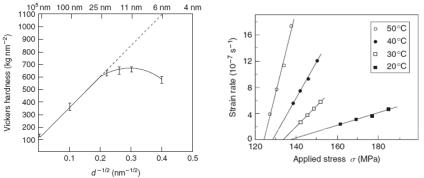
higher than in ordinary polycrystalline solids, causing their density and elastic modulus to be significantly lower.
Fig. 12. Inverse Hall–Petch |
Fig. 13. Flow stress versus strain rate |
behavior in electrodeposited |
in electrodeposited nanocrystalline Cu |
nanocrystalline Ni (Erb, 1995) |
(Cai, Lu and |
|
co-workers, 1999) |
Dislocation activities are heavily confined as the grain size approaches 1 nm, but the yield strength does not increase according to the
Hall–Petch |
relation. Instead, |
|
||||
Hall–Petch relation – соотношение Хол- |
||||||
at grain sizes smaller than |
||||||
ла-Петча |
||||||
about 10 nm, the ‘inverse |
Coble creep type diffusion – диффузион- |
|||||
Hall–Petch’ behavior is ob- |
ная Кобл ползучесть |
|||||
served |
where |
the |
strength |
Newtonian flow behavior – поведение, |
||
drops |
instead |
of rises with |
сходное с ламинарным течением нью- |
|||
decreasing |
|
grain |
size |
тоновской среды (Ньютоновская теку- |
||
|
честь) |
|||||
(fig. 12). |
A |
mechanism |
|
|||
|
dominated by grain boundary activities, possibly Coble creep type diffusion, is thought to be responsible. A related phenomenon is that nanocrystalline metals typically deform with high strain-rate sensitivity (approaching Newtonian flow behavior) once a certain threshold stress is surpassed. Fig. 13 shows the creep results in electrodeposited nanocrystalline copper at low deformation temperatures, where it can be seen that the creep flow stress is given by σ = σo +K˙ε, where σo is a
57

threshold stress, K is a temperature-dependent constant and ˙ε is the strain rate. The linear dependence term K˙ε again suggests a diffusional creep mechanism at low temperatures. Nanocrystalline metals can also exhibit superplasticity (fig. 14), which is a phenomenon characteristic of a high strain-rate sensitivity.
Fig. 14. Superplasticity in electrodeposited nanocrystalline Ni (Mukherjee, 2002)
23. Number the following statements as they occur in the text:
–Glass and carbon fibers are orders of magnitude stronger than their bulk counterparts, and they are highly flexible so they are effective strengtheners and stiffeners in plastic-based composites.
–Compared to fibers, nanotubes and nanowires are even stronger and more flexible, although accurate evaluation of fracture stresses in these materials is difficult.
–The size and time dependence of the elastic-to-plastic transition is unique to small crystals, and is a phenomenon that must be sufficiently understood before the high-strength advantage of small crystals can be utilized in applications.
–The torsional strength of metallic whiskers, as well as the nanoindentation hardness on crystalline specimens, often exhibit a significant rise as the material size decreases
58
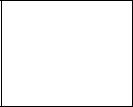
–Despite the impressive strength of small crystal volumes, their plastic deformation following the first yield point is usually very jumpy, exhibiting sporadic, discrete strain bursts.
–The creep deformation of small crystal volumes, even under low temperatures relative to melting, also needs special attention.
–The grain-boundary fraction on nanocrystalline materials is a lot higher than in ordinary polycrystalline solids, causing their density and elastic modulus to be significantly lower.
–Nanocrystalline metals typically deform with high strain-rate sensitivity (approaching Newtonian flow behavior) once a certain threshold stress is surpassed.
–Nanocrystalline metals can also exhibit superplasticity which is a phenomenon characteristic of a high strain-rate sensitivity.
24. Analyse the first of the sentences given below and say whether the underlined word makes the description of the property more or less explicit. Then complete the rest of the sentences with the words intensifying the comparison that you see in the box on your left.
1.The strength of submicron-sized metals and ceramics is considerably higher than their bulk forms.
2.Glass and carbon fibers are _____________________ stronger than their bulk counterparts.
3. Compared to fibers, nanotubes and nanowires are _________ stronger and more flexible.
4. Carbon nanotubes are known to have high fracture strains of 10–30% and
_________________ high Young’s moduli of the order of TPa.
5. Nanocrystalline materials’ grain-boundary fraction is
_________ higher than in ordinary polycrystalline solids, causing their density and elastic modulus to be ________________ lower.
59
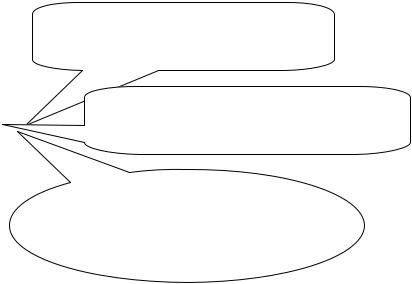
Speaking 4
25. Describe the main mechanical properties of small material volumes. Say how you think they might be useful in different branches of industry: construction, aircraft engineering, production of goods, electronics, etc.
Reading 8
26. You are going to read one more description of nanomaterials focusing on bio-nanotechnology. Before you read it compare a number of definitions of the title term and choose the best from your viewpoint, then read and compare, add detailes.
Bionanotechnology generally refers to the study of how the goals of nanotechnology can be guided by studying how biological "machines" work and adapting these biological motifs into improving existing nanotechnologies or creating new ones.
Bionanotechnology encompasses the study, creation, and illumination of the connections between structural molecular biology and molecular nanotechnology, since the development of nanomachinery might be guided by studying the structure and function of the natural nanomachines found in living cells.
Bionanotechnology is a rapidly advancing area of scientific and technological opportunity that applies the tools and processes of nano/microfabrication to build devices for studying biosystems. Researchers learn from biology to create new micro-nanoscale devices to better understand life processes at the nanoscale.
Bio-nanotechnology
Nanotechnology is increasingly being used in medicine, via biomaterials. These include carbon nanotubes in an ultra-sensitive DNA detector, release of drug molecules controlled by nanoporous membranes
60