
- •Preface
- •Imaging Microscopic Features
- •Measuring the Crystal Structure
- •References
- •Contents
- •1.4 Simulating the Effects of Elastic Scattering: Monte Carlo Calculations
- •What Are the Main Features of the Beam Electron Interaction Volume?
- •How Does the Interaction Volume Change with Composition?
- •How Does the Interaction Volume Change with Incident Beam Energy?
- •How Does the Interaction Volume Change with Specimen Tilt?
- •1.5 A Range Equation To Estimate the Size of the Interaction Volume
- •References
- •2: Backscattered Electrons
- •2.1 Origin
- •2.2.1 BSE Response to Specimen Composition (η vs. Atomic Number, Z)
- •SEM Image Contrast with BSE: “Atomic Number Contrast”
- •SEM Image Contrast: “BSE Topographic Contrast—Number Effects”
- •2.2.3 Angular Distribution of Backscattering
- •Beam Incident at an Acute Angle to the Specimen Surface (Specimen Tilt > 0°)
- •SEM Image Contrast: “BSE Topographic Contrast—Trajectory Effects”
- •2.2.4 Spatial Distribution of Backscattering
- •Depth Distribution of Backscattering
- •Radial Distribution of Backscattered Electrons
- •2.3 Summary
- •References
- •3: Secondary Electrons
- •3.1 Origin
- •3.2 Energy Distribution
- •3.3 Escape Depth of Secondary Electrons
- •3.8 Spatial Characteristics of Secondary Electrons
- •References
- •4: X-Rays
- •4.1 Overview
- •4.2 Characteristic X-Rays
- •4.2.1 Origin
- •4.2.2 Fluorescence Yield
- •4.2.3 X-Ray Families
- •4.2.4 X-Ray Nomenclature
- •4.2.6 Characteristic X-Ray Intensity
- •Isolated Atoms
- •X-Ray Production in Thin Foils
- •X-Ray Intensity Emitted from Thick, Solid Specimens
- •4.3 X-Ray Continuum (bremsstrahlung)
- •4.3.1 X-Ray Continuum Intensity
- •4.3.3 Range of X-ray Production
- •4.4 X-Ray Absorption
- •4.5 X-Ray Fluorescence
- •References
- •5.1 Electron Beam Parameters
- •5.2 Electron Optical Parameters
- •5.2.1 Beam Energy
- •Landing Energy
- •5.2.2 Beam Diameter
- •5.2.3 Beam Current
- •5.2.4 Beam Current Density
- •5.2.5 Beam Convergence Angle, α
- •5.2.6 Beam Solid Angle
- •5.2.7 Electron Optical Brightness, β
- •Brightness Equation
- •5.2.8 Focus
- •Astigmatism
- •5.3 SEM Imaging Modes
- •5.3.1 High Depth-of-Field Mode
- •5.3.2 High-Current Mode
- •5.3.3 Resolution Mode
- •5.3.4 Low-Voltage Mode
- •5.4 Electron Detectors
- •5.4.1 Important Properties of BSE and SE for Detector Design and Operation
- •Abundance
- •Angular Distribution
- •Kinetic Energy Response
- •5.4.2 Detector Characteristics
- •Angular Measures for Electron Detectors
- •Elevation (Take-Off) Angle, ψ, and Azimuthal Angle, ζ
- •Solid Angle, Ω
- •Energy Response
- •Bandwidth
- •5.4.3 Common Types of Electron Detectors
- •Backscattered Electrons
- •Passive Detectors
- •Scintillation Detectors
- •Semiconductor BSE Detectors
- •5.4.4 Secondary Electron Detectors
- •Everhart–Thornley Detector
- •Through-the-Lens (TTL) Electron Detectors
- •TTL SE Detector
- •TTL BSE Detector
- •Measuring the DQE: BSE Semiconductor Detector
- •References
- •6: Image Formation
- •6.1 Image Construction by Scanning Action
- •6.2 Magnification
- •6.3 Making Dimensional Measurements With the SEM: How Big Is That Feature?
- •Using a Calibrated Structure in ImageJ-Fiji
- •6.4 Image Defects
- •6.4.1 Projection Distortion (Foreshortening)
- •6.4.2 Image Defocusing (Blurring)
- •6.5 Making Measurements on Surfaces With Arbitrary Topography: Stereomicroscopy
- •6.5.1 Qualitative Stereomicroscopy
- •Fixed beam, Specimen Position Altered
- •Fixed Specimen, Beam Incidence Angle Changed
- •6.5.2 Quantitative Stereomicroscopy
- •Measuring a Simple Vertical Displacement
- •References
- •7: SEM Image Interpretation
- •7.1 Information in SEM Images
- •7.2.2 Calculating Atomic Number Contrast
- •Establishing a Robust Light-Optical Analogy
- •Getting It Wrong: Breaking the Light-Optical Analogy of the Everhart–Thornley (Positive Bias) Detector
- •Deconstructing the SEM/E–T Image of Topography
- •SUM Mode (A + B)
- •DIFFERENCE Mode (A−B)
- •References
- •References
- •9: Image Defects
- •9.1 Charging
- •9.1.1 What Is Specimen Charging?
- •9.1.3 Techniques to Control Charging Artifacts (High Vacuum Instruments)
- •Observing Uncoated Specimens
- •Coating an Insulating Specimen for Charge Dissipation
- •Choosing the Coating for Imaging Morphology
- •9.2 Radiation Damage
- •9.3 Contamination
- •References
- •10: High Resolution Imaging
- •10.2 Instrumentation Considerations
- •10.4.1 SE Range Effects Produce Bright Edges (Isolated Edges)
- •10.4.4 Too Much of a Good Thing: The Bright Edge Effect Hinders Locating the True Position of an Edge for Critical Dimension Metrology
- •10.5.1 Beam Energy Strategies
- •Low Beam Energy Strategy
- •High Beam Energy Strategy
- •Making More SE1: Apply a Thin High-δ Metal Coating
- •Making Fewer BSEs, SE2, and SE3 by Eliminating Bulk Scattering From the Substrate
- •10.6 Factors That Hinder Achieving High Resolution
- •10.6.2 Pathological Specimen Behavior
- •Contamination
- •Instabilities
- •References
- •11: Low Beam Energy SEM
- •11.3 Selecting the Beam Energy to Control the Spatial Sampling of Imaging Signals
- •11.3.1 Low Beam Energy for High Lateral Resolution SEM
- •11.3.2 Low Beam Energy for High Depth Resolution SEM
- •11.3.3 Extremely Low Beam Energy Imaging
- •References
- •12.1.1 Stable Electron Source Operation
- •12.1.2 Maintaining Beam Integrity
- •12.1.4 Minimizing Contamination
- •12.3.1 Control of Specimen Charging
- •12.5 VPSEM Image Resolution
- •References
- •13: ImageJ and Fiji
- •13.1 The ImageJ Universe
- •13.2 Fiji
- •13.3 Plugins
- •13.4 Where to Learn More
- •References
- •14: SEM Imaging Checklist
- •14.1.1 Conducting or Semiconducting Specimens
- •14.1.2 Insulating Specimens
- •14.2 Electron Signals Available
- •14.2.1 Beam Electron Range
- •14.2.2 Backscattered Electrons
- •14.2.3 Secondary Electrons
- •14.3 Selecting the Electron Detector
- •14.3.2 Backscattered Electron Detectors
- •14.3.3 “Through-the-Lens” Detectors
- •14.4 Selecting the Beam Energy for SEM Imaging
- •14.4.4 High Resolution SEM Imaging
- •Strategy 1
- •Strategy 2
- •14.5 Selecting the Beam Current
- •14.5.1 High Resolution Imaging
- •14.5.2 Low Contrast Features Require High Beam Current and/or Long Frame Time to Establish Visibility
- •14.6 Image Presentation
- •14.6.1 “Live” Display Adjustments
- •14.6.2 Post-Collection Processing
- •14.7 Image Interpretation
- •14.7.1 Observer’s Point of View
- •14.7.3 Contrast Encoding
- •14.8.1 VPSEM Advantages
- •14.8.2 VPSEM Disadvantages
- •15: SEM Case Studies
- •15.1 Case Study: How High Is That Feature Relative to Another?
- •15.2 Revealing Shallow Surface Relief
- •16.1.2 Minor Artifacts: The Si-Escape Peak
- •16.1.3 Minor Artifacts: Coincidence Peaks
- •16.1.4 Minor Artifacts: Si Absorption Edge and Si Internal Fluorescence Peak
- •16.2 “Best Practices” for Electron-Excited EDS Operation
- •16.2.1 Operation of the EDS System
- •Choosing the EDS Time Constant (Resolution and Throughput)
- •Choosing the Solid Angle of the EDS
- •Selecting a Beam Current for an Acceptable Level of System Dead-Time
- •16.3.1 Detector Geometry
- •16.3.2 Process Time
- •16.3.3 Optimal Working Distance
- •16.3.4 Detector Orientation
- •16.3.5 Count Rate Linearity
- •16.3.6 Energy Calibration Linearity
- •16.3.7 Other Items
- •16.3.8 Setting Up a Quality Control Program
- •Using the QC Tools Within DTSA-II
- •Creating a QC Project
- •Linearity of Output Count Rate with Live-Time Dose
- •Resolution and Peak Position Stability with Count Rate
- •Solid Angle for Low X-ray Flux
- •Maximizing Throughput at Moderate Resolution
- •References
- •17: DTSA-II EDS Software
- •17.1 Getting Started With NIST DTSA-II
- •17.1.1 Motivation
- •17.1.2 Platform
- •17.1.3 Overview
- •17.1.4 Design
- •Simulation
- •Quantification
- •Experiment Design
- •Modeled Detectors (. Fig. 17.1)
- •Window Type (. Fig. 17.2)
- •The Optimal Working Distance (. Figs. 17.3 and 17.4)
- •Elevation Angle
- •Sample-to-Detector Distance
- •Detector Area
- •Crystal Thickness
- •Number of Channels, Energy Scale, and Zero Offset
- •Resolution at Mn Kα (Approximate)
- •Azimuthal Angle
- •Gold Layer, Aluminum Layer, Nickel Layer
- •Dead Layer
- •Zero Strobe Discriminator (. Figs. 17.7 and 17.8)
- •Material Editor Dialog (. Figs. 17.9, 17.10, 17.11, 17.12, 17.13, and 17.14)
- •17.2.1 Introduction
- •17.2.2 Monte Carlo Simulation
- •17.2.4 Optional Tables
- •References
- •18: Qualitative Elemental Analysis by Energy Dispersive X-Ray Spectrometry
- •18.1 Quality Assurance Issues for Qualitative Analysis: EDS Calibration
- •18.2 Principles of Qualitative EDS Analysis
- •Exciting Characteristic X-Rays
- •Fluorescence Yield
- •X-ray Absorption
- •Si Escape Peak
- •Coincidence Peaks
- •18.3 Performing Manual Qualitative Analysis
- •Beam Energy
- •Choosing the EDS Resolution (Detector Time Constant)
- •Obtaining Adequate Counts
- •18.4.1 Employ the Available Software Tools
- •18.4.3 Lower Photon Energy Region
- •18.4.5 Checking Your Work
- •18.5 A Worked Example of Manual Peak Identification
- •References
- •19.1 What Is a k-ratio?
- •19.3 Sets of k-ratios
- •19.5 The Analytical Total
- •19.6 Normalization
- •19.7.1 Oxygen by Assumed Stoichiometry
- •19.7.3 Element by Difference
- •19.8 Ways of Reporting Composition
- •19.8.1 Mass Fraction
- •19.8.2 Atomic Fraction
- •19.8.3 Stoichiometry
- •19.8.4 Oxide Fractions
- •Example Calculations
- •19.9 The Accuracy of Quantitative Electron-Excited X-ray Microanalysis
- •19.9.1 Standards-Based k-ratio Protocol
- •19.9.2 “Standardless Analysis”
- •19.10 Appendix
- •19.10.1 The Need for Matrix Corrections To Achieve Quantitative Analysis
- •19.10.2 The Physical Origin of Matrix Effects
- •19.10.3 ZAF Factors in Microanalysis
- •X-ray Generation With Depth, φ(ρz)
- •X-ray Absorption Effect, A
- •X-ray Fluorescence, F
- •References
- •20.2 Instrumentation Requirements
- •20.2.1 Choosing the EDS Parameters
- •EDS Spectrum Channel Energy Width and Spectrum Energy Span
- •EDS Time Constant (Resolution and Throughput)
- •EDS Calibration
- •EDS Solid Angle
- •20.2.2 Choosing the Beam Energy, E0
- •20.2.3 Measuring the Beam Current
- •20.2.4 Choosing the Beam Current
- •Optimizing Analysis Strategy
- •20.3.4 Ba-Ti Interference in BaTiSi3O9
- •20.4 The Need for an Iterative Qualitative and Quantitative Analysis Strategy
- •20.4.2 Analysis of a Stainless Steel
- •20.5 Is the Specimen Homogeneous?
- •20.6 Beam-Sensitive Specimens
- •20.6.1 Alkali Element Migration
- •20.6.2 Materials Subject to Mass Loss During Electron Bombardment—the Marshall-Hall Method
- •Thin Section Analysis
- •Bulk Biological and Organic Specimens
- •References
- •21: Trace Analysis by SEM/EDS
- •21.1 Limits of Detection for SEM/EDS Microanalysis
- •21.2.1 Estimating CDL from a Trace or Minor Constituent from Measuring a Known Standard
- •21.2.2 Estimating CDL After Determination of a Minor or Trace Constituent with Severe Peak Interference from a Major Constituent
- •21.3 Measurements of Trace Constituents by Electron-Excited Energy Dispersive X-ray Spectrometry
- •The Inevitable Physics of Remote Excitation Within the Specimen: Secondary Fluorescence Beyond the Electron Interaction Volume
- •Simulation of Long-Range Secondary X-ray Fluorescence
- •NIST DTSA II Simulation: Vertical Interface Between Two Regions of Different Composition in a Flat Bulk Target
- •NIST DTSA II Simulation: Cubic Particle Embedded in a Bulk Matrix
- •21.5 Summary
- •References
- •22.1.2 Low Beam Energy Analysis Range
- •22.2 Advantage of Low Beam Energy X-Ray Microanalysis
- •22.2.1 Improved Spatial Resolution
- •22.3 Challenges and Limitations of Low Beam Energy X-Ray Microanalysis
- •22.3.1 Reduced Access to Elements
- •22.3.3 At Low Beam Energy, Almost Everything Is Found To Be Layered
- •Analysis of Surface Contamination
- •References
- •23: Analysis of Specimens with Special Geometry: Irregular Bulk Objects and Particles
- •23.2.1 No Chemical Etching
- •23.3 Consequences of Attempting Analysis of Bulk Materials With Rough Surfaces
- •23.4.1 The Raw Analytical Total
- •23.4.2 The Shape of the EDS Spectrum
- •23.5 Best Practices for Analysis of Rough Bulk Samples
- •23.6 Particle Analysis
- •Particle Sample Preparation: Bulk Substrate
- •The Importance of Beam Placement
- •Overscanning
- •“Particle Mass Effect”
- •“Particle Absorption Effect”
- •The Analytical Total Reveals the Impact of Particle Effects
- •Does Overscanning Help?
- •23.6.6 Peak-to-Background (P/B) Method
- •Specimen Geometry Severely Affects the k-ratio, but Not the P/B
- •Using the P/B Correspondence
- •23.7 Summary
- •References
- •24: Compositional Mapping
- •24.2 X-Ray Spectrum Imaging
- •24.2.1 Utilizing XSI Datacubes
- •24.2.2 Derived Spectra
- •SUM Spectrum
- •MAXIMUM PIXEL Spectrum
- •24.3 Quantitative Compositional Mapping
- •24.4 Strategy for XSI Elemental Mapping Data Collection
- •24.4.1 Choosing the EDS Dead-Time
- •24.4.2 Choosing the Pixel Density
- •24.4.3 Choosing the Pixel Dwell Time
- •“Flash Mapping”
- •High Count Mapping
- •References
- •25.1 Gas Scattering Effects in the VPSEM
- •25.1.1 Why Doesn’t the EDS Collimator Exclude the Remote Skirt X-Rays?
- •25.2 What Can Be Done To Minimize gas Scattering in VPSEM?
- •25.2.2 Favorable Sample Characteristics
- •Particle Analysis
- •25.2.3 Unfavorable Sample Characteristics
- •References
- •26.1 Instrumentation
- •26.1.2 EDS Detector
- •26.1.3 Probe Current Measurement Device
- •Direct Measurement: Using a Faraday Cup and Picoammeter
- •A Faraday Cup
- •Electrically Isolated Stage
- •Indirect Measurement: Using a Calibration Spectrum
- •26.1.4 Conductive Coating
- •26.2 Sample Preparation
- •26.2.1 Standard Materials
- •26.2.2 Peak Reference Materials
- •26.3 Initial Set-Up
- •26.3.1 Calibrating the EDS Detector
- •Selecting a Pulse Process Time Constant
- •Energy Calibration
- •Quality Control
- •Sample Orientation
- •Detector Position
- •Probe Current
- •26.4 Collecting Data
- •26.4.1 Exploratory Spectrum
- •26.4.2 Experiment Optimization
- •26.4.3 Selecting Standards
- •26.4.4 Reference Spectra
- •26.4.5 Collecting Standards
- •26.4.6 Collecting Peak-Fitting References
- •26.5 Data Analysis
- •26.5.2 Quantification
- •26.6 Quality Check
- •Reference
- •27.2 Case Study: Aluminum Wire Failures in Residential Wiring
- •References
- •28: Cathodoluminescence
- •28.1 Origin
- •28.2 Measuring Cathodoluminescence
- •28.3 Applications of CL
- •28.3.1 Geology
- •Carbonado Diamond
- •Ancient Impact Zircons
- •28.3.2 Materials Science
- •Semiconductors
- •Lead-Acid Battery Plate Reactions
- •28.3.3 Organic Compounds
- •References
- •29.1.1 Single Crystals
- •29.1.2 Polycrystalline Materials
- •29.1.3 Conditions for Detecting Electron Channeling Contrast
- •Specimen Preparation
- •Instrument Conditions
- •29.2.1 Origin of EBSD Patterns
- •29.2.2 Cameras for EBSD Pattern Detection
- •29.2.3 EBSD Spatial Resolution
- •29.2.5 Steps in Typical EBSD Measurements
- •Sample Preparation for EBSD
- •Align Sample in the SEM
- •Check for EBSD Patterns
- •Adjust SEM and Select EBSD Map Parameters
- •Run the Automated Map
- •29.2.6 Display of the Acquired Data
- •29.2.7 Other Map Components
- •29.2.10 Application Example
- •Application of EBSD To Understand Meteorite Formation
- •29.2.11 Summary
- •Specimen Considerations
- •EBSD Detector
- •Selection of Candidate Crystallographic Phases
- •Microscope Operating Conditions and Pattern Optimization
- •Selection of EBSD Acquisition Parameters
- •Collect the Orientation Map
- •References
- •30.1 Introduction
- •30.2 Ion–Solid Interactions
- •30.3 Focused Ion Beam Systems
- •30.5 Preparation of Samples for SEM
- •30.5.1 Cross-Section Preparation
- •30.5.2 FIB Sample Preparation for 3D Techniques and Imaging
- •30.6 Summary
- •References
- •31: Ion Beam Microscopy
- •31.1 What Is So Useful About Ions?
- •31.2 Generating Ion Beams
- •31.3 Signal Generation in the HIM
- •31.5 Patterning with Ion Beams
- •31.7 Chemical Microanalysis with Ion Beams
- •References
- •Appendix
- •A Database of Electron–Solid Interactions
- •A Database of Electron–Solid Interactions
- •Introduction
- •Backscattered Electrons
- •Secondary Yields
- •Stopping Powers
- •X-ray Ionization Cross Sections
- •Conclusions
- •References
- •Index
- •Reference List
- •Index
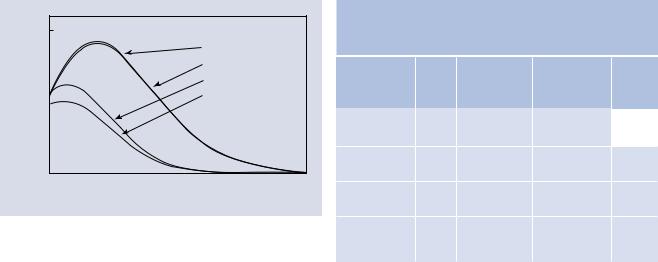
19.10· Appendix
2.5
|
|
|
|
|
|
|
|
|
|
|
AI Kα in AI-3 wt% Cu |
|||||
|
2.0 |
|
|
|
|
|
|
|
|
|
AI Kα in AI |
|
|
|||
|
|
|
|
|
|
|
|
|
|
|
|
|||||
(rz) |
1.5 |
|
|
|
|
|
|
|
|
|
Cu Kα in Cu |
|
|
|||
|
|
|
|
|
|
|
|
|
Cu Kα in Al-3 wt% Cu |
|||||||
|
|
|
|
|
|
|
|
|
||||||||
|
|
|
|
|
|
|
|
|
|
|||||||
f |
|
|
|
|
|
|
|
|
|
|
|
|
|
|
|
|
|
1.0 |
|
|
|
|
|
|
|
|
|
|
|
|
|
|
|
|
|
|
|
|
|
|
|
|
|
|
|
|
|
|
|
|
|
0.5 |
|
|
|
|
|
|
|
|
|
|
|
|
|
|
|
|
|
|
|
|
|
|
|
|
|
|
|
|
|
|
|
|
|
0.00 |
|
|
|
|
|
|
|
|
|
|
|
|
|
|
|
|
|
|
|
|
|
|
|
|
|
|
|
|
|
|
||
|
100 |
200 |
300 |
400 |
500 |
600 |
700 |
|||||||||
|
|
|
|
|
Mass-depth (ρz) (10-6g/cm2) |
|
|
|
|
. Fig. 19.10 Calculated φ(ρz) curves for Al K-L3 and Cu K-L3 in Al, Cu, and Al-3wt%Cu at E0 = 15 keV; calculated using PROZA
303 |
|
19 |
|
|
|
. Table 19.3 Generated X-ray intensities in Al, Cu, and Al-3wt%Cu alloy, as calculated with PROZA (Bastin and Heijligers 1990, 1991)
Sample |
X-ray |
φ(ρz)i,gen |
Atomic |
φ0 |
|
|
Area (cm2/g) |
number |
|
|
|
|
factor, Zi |
|
|
|
3.34 × 10−4 |
|
|
Cu |
Cu |
1.0 |
1.39 |
|
|
K-L3 |
|
|
|
Al |
Al |
7.85 × 10−4 |
1.0 |
1.33 |
|
K-L3 |
|
|
|
Al-3wt%Cu |
Cu |
2.76 × 10−4 |
0.826 |
1.20 |
|
K-L3 |
|
|
|
Al-3wt%Cu |
Al |
7.89 × 10−4 |
1.005 |
1.34 |
|
K-L3 |
|
|
|
curve in the alloy is smaller than that of pure Cu because the average atomic number of the Al – 3 wt % Cu sample is so much lower, almost the same as pure Al. In this case, less backscattering of the primary high energy electron beam occurs and fewer Cu K-L3 X-rays are generated. On the other hand the Al K-L3 φ(ρz) curves for the alloy and the pure element are essentially the same since the average atomic number of the specimen is so close to that of pure Al. Although the variation of φ(ρz) curves with atomic number and initial operating energy is complex, a knowledge of the pertinent X-ray generation curves is critical to understanding what is happening in the specimen and the standard for the element of interest.
The generated characteristic X-ray intensity, Ii gen, for each element, i, in the specimen can be obtained by taking the area under the φ(ρz) versus ρz curve, that is, by summing the values of φ(ρz) for all the layers (ρz) in mass thickness within the specimen for the X-ray of interest. We will call this area “φ(ρz)i,gen Area.” . Table 19.3 lists the calculated values, using the PROZA program, of the φ(ρz)i,gen Area for the 15 kev φ(ρz) curves shown in . Fig. 19.10 (Cu K-L3 and Al K-L3 in the pure elements and Cu K-L3 and Al K-L3 in an alloy of Al – 3 wt % Cu and the corresponding
values of φ0). A comparison of the φ(ρz)i,gen Area values for Al K-L3 in Al and in the Al – 3 wt % Cu alloy shows very
similar values while a comparison of the φ(ρz)i,gen Area values for Cu K-L3 in pure Cu and in the Al – 3 wt % Cu alloy shows that about 17 % fewer Cu K-L3 X-rays are generated in the alloy. The latter variation is due to the different atomic numbers of the pure Cu and the Al – 3 wt% Cu alloy specimen. The different atomic number matrices cause a change in φ0 (see . Table 19.3) and the height of the φ(ρz) curves.
The atomic number correction, Zi, can be calculated by
taking the ratio of φ(ρz)i,gen Area for the standard to φ(ρz)i,gen Area for element i in the specimen. Pure Cu and pure Al are
the standards for Cu K-L3 and Al K-L3 respectively. The values of the calculated ratios of generated X-ray intensities, pure element standard to specimen (Atomic number effect, ZAl, ZCu) are also given in . Table 19.3 As discussed above, it is expected that the atomic number correction for a heavy element (Cu) in a light element matrix (Al – 3 wt % Cu) is
less than 1.0 and the atomic number correction for a light element (Al) in a heavy element matrix (Al – 3 wt % Cu) is greater than 1.0. The calculated data in . Table 19.3 also show this relationship.
In summary, the atomic number matrix correction, Zi, is
equal to the ratio of Zi,std in the standard to Zi,unk in the unknown. Using appropriate φ(ρz) curves, correction Zi can
be calculated by taking the ratio of Igen,std for the standard to Igen,unk for the unknown for each element, i, in the sample. It is important to note that the φ(ρz) curves for multi-element
samples and elemental standards which can be used for the calculation of the atomic number effect inherently contain the R and S factors discussed previously.
X-ray Absorption Effect, A
. Figure 19.11 illustrates the effect of varying the initial electron beam energy using Monte Carlo simulations on the positions where K-shell X-ray generation occurs for Cu at three initial electron energies, 10, 20, and 30 keV. This figure shows that the Cu characteristic X-rays are generated deeper in the specimen and the X-ray generation volume becomes larger as E0 increases. From these plots, we can see that the sites of inner shell ionizations which give rise to characteristic X-rays are created over a range of depth below the surface of the specimen.
Created over a range of depth, the X-rays will have to pass through a certain amount of matter to reach the detector, and as explained in 7 Chapter 4 (X-rays), the photoelectric absorption process will decrease the intensity. It is important to realize that the X-ray photons are either absorbed or else they pass through the specimen with their original energy unchanged, so that they are still characteristic of the atoms which emitted the X-rays. Absorption follows an exponential law, so as X-rays are generated deeper in the specimen, a progressively greater fraction is lost to absorption.
From the Monte Carlo plots of . Fig. 19.11, one recognizes that the depth distribution of ionization is a complicated function. To quantitatively calculate the effect of X-ray absorption, an accurate description of the X-ray distribution in depth is
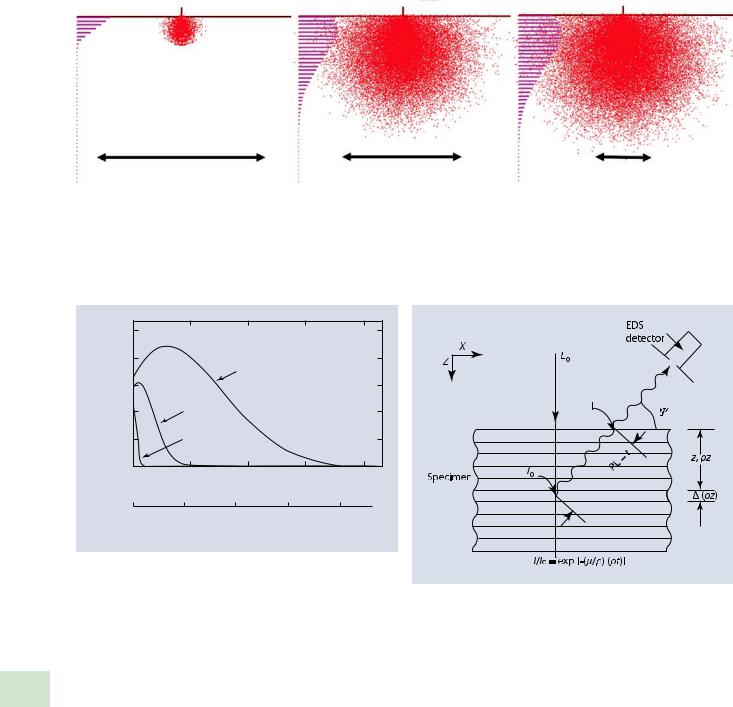
304\ Chapter 19 · Quantitative Analysis: From k-ratio to Composition
E0 = 10 keV |
E0 = 20 keV |
E0 = 30 keV |
0.5 µm |
1 µm |
1 µm |
Cu
K-L3 = generation ϕ(ρz) = distribution
. Fig. 19.11 Monte Carlo simulations (Joy Monte Carlo) of the X-ray generation volume for Cu K-L3at E0 = 10 keV, 20 keV and 30 keV. The sites of X-ray generation (red dots) are projected on the x-z plane, and the resulting φ(ρz) distribution is shown
. Fig. 19.12 Calculated φ(ρz) curves for Cu K-L3 in Cu at E0 = 10 keV, 20 keV, and 30 keV; calculated using PROZA
needed. Fortunately, the complex three-dimensional distribution can be reduced to a one-dimensional problem for the calculation of absorption, since the path out of the specimen towards the X-ray detector only depends on depth. The
19 φ(ρz) curves discussed previously give the generated X-ray distribution of X-rays in depth (See . Figs. 19.8, 19.9, and 19.10). . Figure 19.12 shows calculated φ(ρz) curves for Cu K-L3 X-rays in pure Cu for initial beam energies of 10, 15, and 30 keV. The curves extend deeper (in mass depth or depth) in the sample with increasing E0. The φ0 values also increase with increasing initial electron beam energies since the energy of the backscattered electrons increases with higher values of E0.
The X-rays which escape from any depth can be found by placing the appropriate path length in the X-ray absorption equation for the ratio of the measured X-ray intensity,
I, to the generated X-ray intensity at some position in the sample, I0:
. Fig. 19.13 Schematic diagram of absorption in the measurement or calculation of the φ(ρz) curve for emitted X-rays. PL = path length, ψ = X-ray take-off angle (detector elevation angle above surface)
|
0 |
( |
|
)( |
|
) |
|
|
I / I |
|
= exp − |
µ / ρ |
|
ρt |
|
\ |
(19.18) |
|
|
|
|
|
|
|
|
The terms in the absorption equation are (μ/ρ), the mass absorption coefficient; ρ, the specimen density; and t, the path length (PL) that the X-ray traverses within the specimen before it reaches the surface, z = ρz = 0. For the purpose of our interests, I represents the X-ray intensity which leaves the surface of the sample and I0 represents the X-ray intensity generated at some position within the X-ray generation volume. Since the X-ray spectrometer is usually placed at an acute angle from the specimen surface, the so-called take-off angle, ψ, the path length from a given depth z is given by PL = z csc ψ, as shown in . Fig. 19.13. When this correction for absorption is applied to each of the many layers (ρz) in

19.10 · Appendix
. Fig. 19.14 Calculated generated and emitted φ(ρz) curves for Al K-L3 in a Cu matrix at E0 = 20 keV
305 |
|
19 |
|
|
|
3.0
2.0 |
AI Kα in Cu |
|
(generated) |
||
|
f (rz)
1.0
AI Kα in Cu (emitted)
0.0
0 |
100 |
200 |
300 |
400 |
500 |
600 |
700 |
Mass-depth (ρz) (10-6g/cm2)
the φ(ρz) curve, a new curve results, which gives the depth distribution of emitted X-rays. An example of the generated and emitted depth distribution curves for Al K-L3 at an initial electron beam energy of 15 keV (calculated using the PROZA program (Bastin and Heijligers 1990, 1991)) is shown in . Fig. 19.14 for a trace amount (0.1 wt%) of Al in a pure copper matrix. The area under the φ(ρz) curve represents the X-ray intensity. The difference in the integrated area between the generated and emitted φ(ρz) curves represents the total X-ray loss due to absorption. The absorption correction factor in quantitative matrix corrections is calculated on the basis of the φ(ρz) distribution. . Figure 19.5, for example, illustrates the large amount of Ni K-L3 absorbed in the Fe-Ni alloy series as a function of composition.
X-ray absorption is usually the largest correction factor that must be considered in the measurement of elemental composition by electron-excited X-ray microanalysis. For a given X-ray path length, the mass absorption coefficient, (μ/ρ), for each measured characteristic X-ray peak controls the amount of absorption. The value of (μ/ρ) varies greatly from one X-ray to another and is dependent on the matrix elements of the specimen (see 7 Chapter 4, “X-rays”). For example, the mass absorption coefficient for Fe K-L3 radiation in Ni is 90.0 cm2/g, while the mass absorption coefficient for Al K-L3 radiation in Ni is 4837 cm2/g. Using Eq. (19.18) and a nominal path length of 1 μm in a Ni sample containing small amounts of Fe and Al, the ratio of X-rays emitted at the sample surface to the X-rays generated in the sample, I/I0, is 0.923 for Fe K-L3 radiation but only 0.0135 for Al K-L3 radiation. In this example, Al K-L3 radiation is very heavily absorbed with respect to Fe K-L3 radiation in the Ni sample. Such a large amount of absorption must be taken account of in any quantitative X-ray analysis scheme. Even more serious effects of absorption occur when considering
the measurement of the light elements, for example, Be, B, C, N, O, and so on. For example, the mass absorption coefficient for C K-L radiation in Ni is 17,270 cm2/g, so large that in most practical analyses, no C K-L radiation can be measured if the absorption path length is 1 μm. Significant amounts of C K-L radiation can only be measured in a Ni sample within 0.1 μm of the surface. In such an analysis situation, the initial electron beam energy should be held below 10 keV so that the C K-L X-ray source is produced close to the sample surface.
As shown in . Fig. 19.12, X-rays are generated up to several micrometers into the specimen. Therefore the X-ray path length (PL = t) and the relative amount of X-rays available to the X-ray detection system after absorption (I/I0) vary with the depth at which each X-ray is generated in the specimen. In addition to the position, ρz or z, at which a given X-ray is generated within the specimen, the relation of that depth to the X-ray detector is also important since a combination of both factors determine the X-ray path length for absorption. . Figure 19.15 shows the geometrical relationship between the position at which an X-ray is generated and the position of the collimator which allows X-rays into the EDS detector. If the specimen is normal to the electron beam (. Fig. 19.15), the angle between the specimen surface and the direction of the X-rays into the detector is the take-off angle ψ. The path length, t = PL, over which X-rays can be absorbed in the sample is calculated by multiplying the depth in the specimen, z, where the X-ray is generated, by the cosecant (the reciprocal of the sine), of the take-off angle, ψ. A larger take-off angle will yield a shorter path length in the specimen and will minimize absorption.
The path length can be further minimized by decreasing the depth of X-ray generation, Rx, that is by using the minimum electron beam energy, E0, consistent with the excitation of
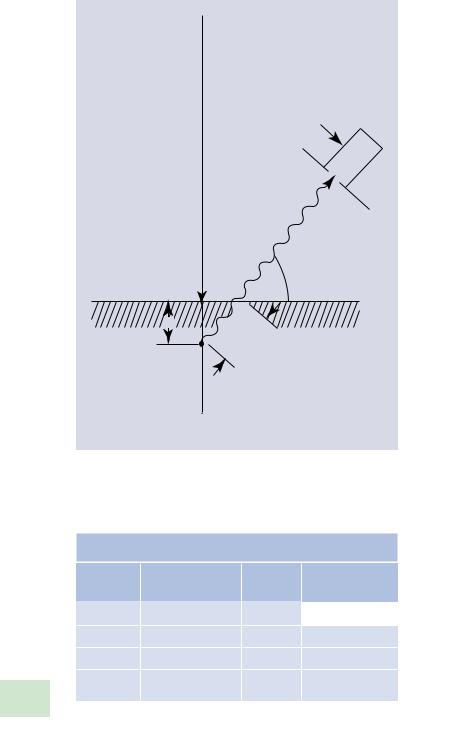
\306 Chapter 19 · Quantitative Analysis: From k-ratio to Composition
Eo
EDS
Detector
Ψ
Z |
t |
|
= |
|
PL |
Specimen
PL = Z COSEC Ψ
. Fig. 19.15 Schematic diagram showing the X-ray absorption path length in a thick, flat-polished sample: PL = absorption path length; ψ = X-ray take-off angle (detector elevation angle above surface)
. Table 19.4 Path Length, PL, for Al K-L3 X-rays in Al
E0 |
Take-Off Angle, ψ |
Rx (μm) |
Path Length, PL, |
|
|
|
(μm) |
|
|
|
|
10 |
15 |
0.3 |
1.16 |
|
|
|
|
10 |
60 |
0.3 |
0.35 |
30 |
15 |
2.0 |
7.7 |
30 |
60 |
2.0 |
2.3 |
19
the X-ray lines used for analysis. . Table 19.4 shows the variation of the path length that can occur if one varies the initial electron beam energy for Al K-L3 X-rays in Al from 10 to 30 keV and the take-off angle, ψ = 15° and ψ = 60°.
The variation in PL is larger than a factor of 20, from 0.35 μm at the lowest keV and highest take-off angle to 7.7 μm at the highest keV and lowest take-off angle. Clearly the analyst’s choices of the initial electron beam energy and the X-ray take-off angle have a major effect on the path length and therefore the amount of absorption that occurs.
In summary, using appropriate formulations for X-ray generation with depth or φ(ρz) curves, the effect of absorption can be obtained by considering absorption of X-rays from element i as they leave the sample. The absorption correction, Ai, can be calculated by taking the ratio of the effect of
absorption for the standard, Ai,std, to X-ray absorption for the unknown, Ai,unk, for each element, i, in the sample. The effect of absorption can be minimized by decreasing the path length
of the X-rays in the specimen through careful choice of the initial beam energy and by selecting, when possible, a high take-off angle.
X-ray Fluorescence, F
Photoelectric absorption results in the ionization of inner atomic shells, and those ionizations can also cause the emission of characteristic X-rays. For fluorescence to occur, an atom species must be present in the target which has a critical excitation energy less than the energy of the characteristic X-rays being absorbed. In such a case, the measured X-ray intensity from this second element will include both the direct electron-excited intensity as well as the additional intensity generated by the fluorescence effect. Generally, the fluorescence effect can be ignored unless the photon energy is less than 5 keV greater than the critical excitation energy, Ec.
The significance of the fluorescence correction, Fi, can be illustrated by considering the binary system Fe-Ni. In this system, the Ni K-L3 characteristic energy at 7.478 keV is greater than the energy for excitation of Fe K radiation, Ec =7.11 keV. Therefore, an additional amount of Fe K-L3 radiation is produced beyond that due to the direct beam on Fe.
. Figure 19.5 shows the effect of fluorescence in the Fe-Ni system at an initial electron beam energy of 30 keV and a take-off angle, ψ, of 52.5°. Under these conditions, the atomic number effect, ZFe, and the absorption effect, AFe, for Fe K-L3 are very close to 1.0. The measured kFe ratio lies well above the first approximation straight line relationship. The additional intensity is given by the effect of fluorescence. As an example, for a 10 wt% Fe – 90 wt% Ni alloy, the amount of iron fluorescence is about 25%.
The quantitative calculation of the fluorescence effect requires a knowledge of the depth distribution over which the characteristic X-rays are absorbed. The φ(ρz) curve of elec- tron-generated X-rays is the starting point for the fluorescence calculation, and a new φ(ρz) curve for X-ray-generated X-rays is determined. The electron-generated X-rays are emitted isotropically. From the X-ray intensity generated in each of the layers (ρz) of the φ(ρz) distribution, the calculation next considers the propagation of that radiation over a spherical volume centered on the depth ρz of that layer, calculating the absorption based on the radial distance from the starting layer and determining the contributions of absorption to each layer (ρz) in the X-ray-induced φ(ρz) distribution. Because of the longer range of X-rays than electrons in materials, the X-ray- induced φ(ρz) distribution covers a much greater depth, generally an order of magnitude or more than the electron-induced