
01 POWER ISLAND / 02 H2+NH3 / Royal Swiss 2024 Techno-Economic Analysis of Wind Power-to-Hydrogen
.pdf
CHAPTER 4. GÄVLE HARBOR
Gävle harbor is the largest logistics hub in mid Sweden, where six million tons of goods are annually handled. Its infrastructure consists of six different terminals, including bulk, container, energy, and combined terminal. These terminals are managed by various specialized companies and are daily visited by large transport ships and trains. The operations taking place in the harbor include, among other things, the export of the region’s industrial production of steel, wood, and paper, while simultaneously importing raw materials for industry, consumer products, fuel, and project cargoes [75].
Gävle harbor has the aim to contribute to the global transition process of implementing green operating methods. Specifically, the Gävle harbor has planned to execute a 10-year program called “Energy-Optimized Port Cluster 2030”, where the main mission is to reduce CO2-emissions and promote energy efficiency, both at national and regional level in accordance with the Paris Agreement [75]. This program includes the entire operations taking place in the port, and therefore will be carried out in a collaboration with the companies present in the port. Some of the actions that will take place in the program are [75]:
Sustainable development of the port – implementing methods that yield in enhanced energy efficiency and increase the usage of electrically powered vehicles.
Electrify the railways that are directly connected to the terminals – infrastructure investment by the Swedish Transport Agency.
Sustainable cargo management – the container terminal’s cranes will either be operating on fossil-free fuels such as green hydrogen or electrified.
Green transportation – the use of green fuels when transporting goods from the
region’s industries to the port. The aim is to reduce CO2-emissions from the heavy truck traffic.
Furthermore, Gävle harbor AB is also actively engaging in the development of regional hydrogen infrastructure. The port has planned to dedicate a “green refueling zone”, where fossil-free fueled operating trucks can visit and refill the tank [75]. As previously mentioned, the company Svea Vind Offshore is currently in the process of developing a grid-powered hydrogen production facility in the harbor. In the future, this company also plans to construct an offshore wind plant right outside of the harbor, and Mattias Wärn, CEO of Svea Vind Offshore, in an article says that from the perspective of hydrogen production, this could mean a significant increase in production scale [76].
4.1 Vehicles Operating in Gävle Harbor
This section aims to provide an insight into the various types of vehicles operating in the port, and the methodology employed to estimate the respective diesel consumption. The purpose is to apply equation (10) to calculate the corresponding hydrogen consumption. Based on this, information about the harbor’s hypothetical daily hydrogen demand can be computed.
49

4.1.1 Trucks
The haulage contractors in the harbor, have reported that an average of 202 specific trucks are operating in the port every day, where each is estimated to consume about 230 l diesel/day. This information can be used to determine the corresponding hydrogen consumption by applying equation (10), with the specific variable’s values listed in Table 25. The calculated corresponding hydrogen consumption of these trucks is available in Appendix E, Table 58.
Table 25. Data of diesel engine, Fuel cell, and the corresponding fuels [19].
|
Variable |
Value |
|
|
|
|
|
|
ηdiesel |
|
|
|
45 [%] |
||
|
ηH2 |
60 [%] |
|
|
Ediesel |
9.8 |
[kWh/l] |
|
EH2 |
33.33 |
[kWh/kg] |
|
|
|
4.1.2 Machines
The loading machines operating in the harbor are majority owned by Yilport, while some belongs to Sören Thyr AB. The quantity and types of machines operating in the terminals are listed in Table 26 [19].
Table 26. Specific Data and Information Regarding the Machines Operating in Gävle Habor [19].
Machines |
Lift Capacity |
Quantity |
Operator |
|
|
|
|
|
|
|
|
Forklifts |
2–33 (average 9.52) [tons] |
43 |
Yilport |
Reachstackers |
45 [tons] |
14 |
Yilport |
Wheel Loaders |
2–22 (average 9.75) [tons] |
14 |
Yilport |
Terminal Tractors |
32 [tons] |
11 |
Yilport |
|
|
|
|
|
|
|
|
Forklifts |
unknown |
10 |
Sören Thyr AB |
Reachstackers |
unknown |
4 |
Sören Thyr AB |
Wheel Loaders |
unknown |
4 |
Sören Thyr AB |
Terminal Tractors |
unknown |
7 |
Sören Thyr AB |
|
|
|
|
In contrast to the calculation of the trucks’ hydrogen consumption, some of the assumptions about the machines needs to be made prior to calculation of the diesel consumption. This includes considering which type of fuel is being used due to some machines are HVO-fueled. In this report, it is assumed that all machines are operating on diesel fuel. Furthermore, certain machines in the harbor, such as small forklifts, are already electrically powered. However, the number of electrified forklifts in the port are unavailable. This means that the actual number of forklifts needing a transition to green fuel is lower than what is specified in Table 26. The sizes of Sören Thyr AB’s machines were unknown, therefore, these machines will henceforth be assumed to have the similar sizes as Yilport’s machines [19].
Technical information about the machines were limited, due to some manufacturers being unknown and therefore assumptions were made. The manufacturer Kalmar’s product catalogue was used as a reference to estimate the diesel consumption of reachstackers and forklifts (lift capacity larger than 5 tons) [19]. This is due to around 35% of Yilport’s machines were from the manufacturer Kalmar. The performance data of the reachstackers in the Kalmar catalogue were
50
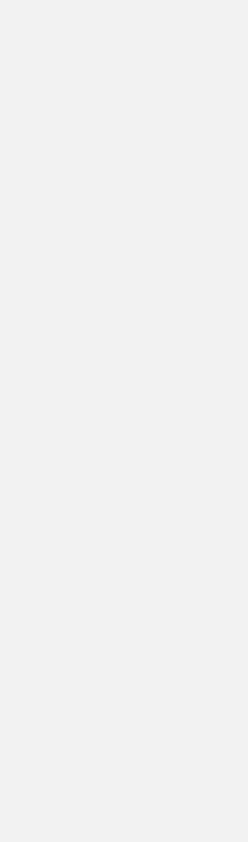
presented in ranges, where the mean value was used to compute the diesel consumption. Apart from the manufacturer Kalmar, technical information could also be obtained from manufacturer Linde as 13% of Yilport’s machines were from there. This information was used to compute the diesel consumption of forklifts with a lift capacity of lower than 5 tons [19].
The diesel-fueled Kalmar machines are equipped with diesel engines that were manufactured by Volvo. The engine efficiency can be estimated to be around 50% [19]. This value was assumed in all calculations of hydrogen consumption when implementing equation (10). Technical data about the diesel and corresponding hydrogen consumption for the forklifts and reachstackers, are available in Appendix D, Table 59 and 60, respectively [19].
Moreover, estimation of the wheel loaders’ diesel consumption required additional assumptions in terms of the drivers’ operating behavior. That is the diesel consumption of such vehicles were directly influenced by how these vehicles were operated by the driver [19]. Technical information regarding the diesel consumption of the wheel loaders were obtained from [19], which provided data about various models’ fuel consumption based on different workloads (low, medium, or high). In this report, the wheel loaders were assumed to operate at a medium load. The diesel consumption and the corresponding hydrogen consumption of the wheel loaders could be calculated and are available in Table 61, in Appendix E [19].
Technical information about the terminal tractors were obtained from [19]. According to this source, terminal tractors were estimated to consume about 4.55 l diesel/h. This value was applied to the terminal tractor of Gävle harbor, and the computed hydrogen consumption is listed in Table 62 [19]. To estimate the daily hydrogen consumption of these machines, it was assumed that a standard working day in the harbor consist of 8 hours for a whole year (365 days).
4.1.3 Transition Process
The transition process from diesel fueled to green hydrogen fueled vehicles is expected to occur gradually. Therefore, three different hydrogen transition stages are proposed: 15%, 50% and 100% of the daily hydrogen demand required to operate the trucks and machines of the Gävle harbor. The final stage represents a fully green-fueled operating harbor, where the PtH plant is capable to provide 100% of the daily hydrogen demand. Furthermore, these transition stages will serve as a foundation to estimate within which range the hydrogen produced from the three different facility sizes, would fall within. The daily hydrogen demand of the respective transition stage is presented in Table 27. This table is based on the vehicle composition listed in Table 63, in Appendix E.
Table 27. Daily hydrogen demand based on transition stages [19].
Transition Stage |
|
Value |
|
|
|
|
|
|
|
|
|
15 |
[%] |
1808 |
[kg/day] |
50 |
[%] |
6025 |
[kg/day] |
100 |
[%] |
12050 |
[kg/day] |
|
|
|
|
51
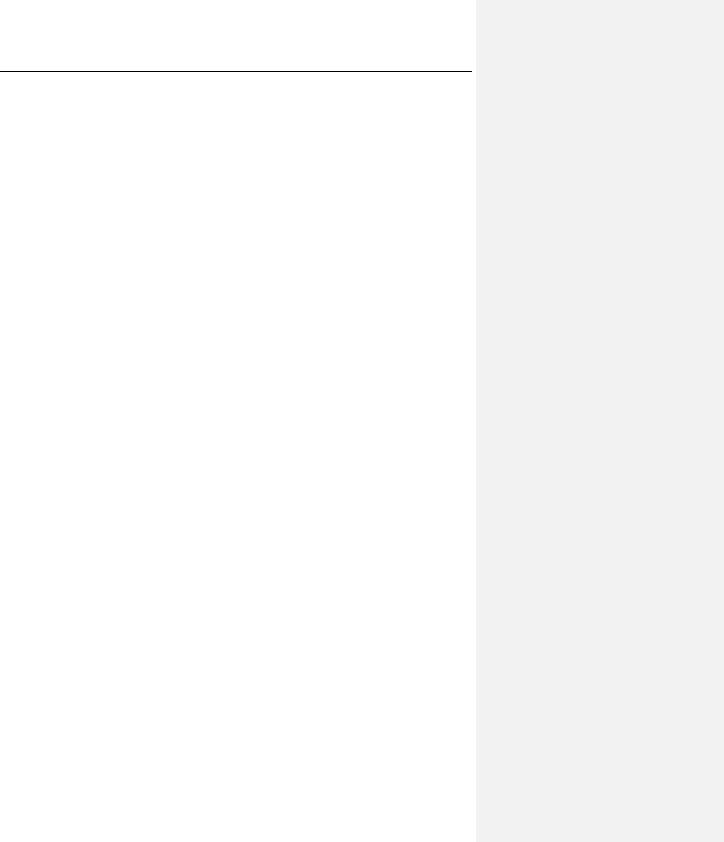
CHAPTER 5. METHODOLOGY
This chapter aims to provide an insight into the methods employed to analyze the case study. This involves describing the process of extracting and processing relevant data and information about each component in the PtH plant from. A flowchart will also be developed to illustrate the process of producing hydrogen from wind energy. This flowchart will serve as a foundation to formulate and express the operational processes of respective component in the PtH plant using mathematical equations.
5.1 Pre-Processing and Transformation of Data
This section will explain the process of re-calculating and pre-processing the gathered information and data in order to adjust and simulate the PtH plant’s operation according to the study case. As previously mentioned, the simulations of the PtH park will be carried out by implementing two different strategies. The first step is described in section 5.1.1, which involves simulating power production of the wind plant using MATLAB. The second step is described in section 5.1.2, and it involves producing hydrogen based on predetermined conditions using Python. Once accomplished, the resulting outcome will be integrated into two different economic modeling tools, described in section 5.2, for further analysis.
5.1.1 Electricity Production from Wind Farm
To simulate the wind power production in MATLAB, hourly wind speed data for the years 2019, 2020, and 2021 were collected from the wind station Eggegrund A [62]. It was discovered that 38 out of 8 760 wind speed hours were missing. The majority of these missing data points were spread out over the year and could simply be computed by interpolating the closest data points. However, in some instances, there were sequences of missing wind data. These data points could be calculated by combining the wind data measured by the nearby wind station, Utvalnäs Aut, with
Eggegrund A’s data points that were closest to the sequence gap, also using interpolation.
Additionally, the average wind speed in the vicinity of Eggegrund A, is available in Table 28.
Table 28. Average Wind Speed at Eggegrund A [62].
Year |
Average Wind Speed |
|
|
|
|
2019 |
5.39 [m/s] |
2020 |
4.41 [m/s] |
2021 |
5.56 [m/s] |
|
|
The MATLAB code used to simulate power production throughout a year, is available in Appendix A. The code utilizes a linear interpolation to produce power output as a function of wind speed, with Lillgrund being used as a reference.
5.1.1.1 Wind Farm Connected to Grid
A wind farm located in the northern region of Sweden is integrated into the grid via a connecting point. The farm’s geographical location and its electricity input into the grid can be used to identify and quantify the varying expenses (WGcost) [€/MWh] and varying incomes (WGinc) [€/MWh]. The expenses involve the cost of supplying electricity to the grid (energy fee), while the income is
52

generated from the sales of electricity and electricity certificate. These are expressed in equations 14 and 15, respectively.
WGcost = Energy Fee |
(14) |
WGinc = Spot price + Certificate |
(15) |
The total generated income can be obtained by subtracting (14) from (15) as seen in equation 16.
WG3 = WGinc − WGcost |
(16) |
WG3 in equation (16) is the alternative income obtained if the electricity would have been sold to the grid instead of powering the hydrogen production facility. Therefore, equation (16) will be integrated into the calculations of the break-even spot prices in equation (30), as the opportunity cost.
5.1.2 Hydrogen Production Facility
In this section, the operational processes of the main equipment are derived from the flowchart visualized in Figure 23 and expressed in the form of operational equations as described in section 5.1.2.1–5.1.2.3. The operational equations for each unit are then converted into variable cost equations. Moreover, in the hydrogen production simulation, factors such as the spot price, target year, generated wind power and electrolyzer capacity, are considered. The Python code utilized to simulate the H2 production is available in Appendix B.
Figure 23. A simplified flowchart of the H2 production facility’s operational process.
5.1.2.1 Variable Operational Process of Electrolyzer
In this thesis, the variable operation (VOElec) for all electrolyzer units includes the following two
two parameters; power (Epower) (MWh/kg H2) and water (Wcons) (kg H2O(l)/kg H2) consumptions. This is due to electricity and water being the only feedstocks to the water electrolyzer as seen in Figure 23. The operational cost of an electrolyzer can be obtained by linking its consumption cost
of power (Epower) (MWh/kg H2) and water (Wcons) (kg H2O(l)/kg H2) to the spot price (€/MWh) and water cost (CW) (€/kg H2O), respectively. However, since this report involves operating the hydrogen production site at profitable production hours, the power consumption of electrolyzer
(Epower) (MWh/kg H2) will be linked to equation (16) as expressed in the equations (18) and (19). This is due to the aim of finding spot prices where it is more profitable to produce hydrogen over selling the electricity to the grid.
EH2 = EPower ∙ WG3 |
(18) |
Wwater = Wcons ∙ CW |
(19) |
53

Where EH2 (€/kg H2), Wwater (€/kg H2) and WG3 (€/MWh) are the variable cost of power consumption, water consumption and the opportunity cost of alternative two, respectively.
Additional variable costs such as energy tax (EEn,tax) (€/MWh) for using electricity to produce hydrogen, is also considered. Thus, the total variable cost (€/kg H2) of an electrolyzer can be
expressed according to equation (20).
VCElec = EH2 + Wwater + EEn,tax ∙ EPower |
(20) |
The equation (20) can be re-written to equation (21). |
|
VCElec = EPower ∙ WG3 + Wcons ∙ CW + EEn,tax ∙ EPower |
(21) |
However, since EEn,tax = 0 (EUR/MWh) for an electrolyzer, the equation (21) can be reduced to equation (22).
VCElec = EPower ∙ WG3 + Wcons ∙ CW |
(22) |
Where VCElec (€/kg H2) is an expression for the electrolyzer’s total variable cost.
5.1.2.2 Variable Operational Process of Compressor
The variable cost of a compressor (VCcomp) can be derived from the shaft power (Cpower) (MWh/kg H2) required to compress the hydrogen until a specific pressure is obtained. Therefore, the variable operational cost includes the cost of electricity (€/MWh) that is consumed by the compressor. Therefore, the alternative profit, WG3 (€/MWh), is also considered here and since the hydrogen production site will only operate at profitable hours; the compressor’s electricity consumption (CH2 ) (€/kg H2) includes the shaft power being linked to equation (16) as seen in
equation (24). Additional costs can also be energy tax (EEn,tax) (€/MWh) due to using electricity for hydrogen compression as seen in equation (25).
CH2 = Cpower ∙ WG3 |
(24) |
Energy tax = EEn,tax ∙ Cpower |
(25) |
Where the WG3 (€/MWh) is the alternative income of alternative two.
The equations (24) and (25) can be summed to equation (26) to obtain an expression of the total operational cost (€/kg H2) of a compressor.
VCcomp = Cpower ∙ WG3 + EEn,tax ∙ Cpower |
(26) |
5.1.2.3 Total Variable Operational Process of Hydrogen Facility
The total variable cost (VCtot) (€/kg H2) can be written as the sum of the equation (22) and (26) as shown in (28).
VCtot = VCElec + VCcomp |
(28) |
Where equation (28) can be re-written to equation (29), which represents the total variable cost (VCtot) (€/kg H2) of the hydrogen production facility in this case study.
54
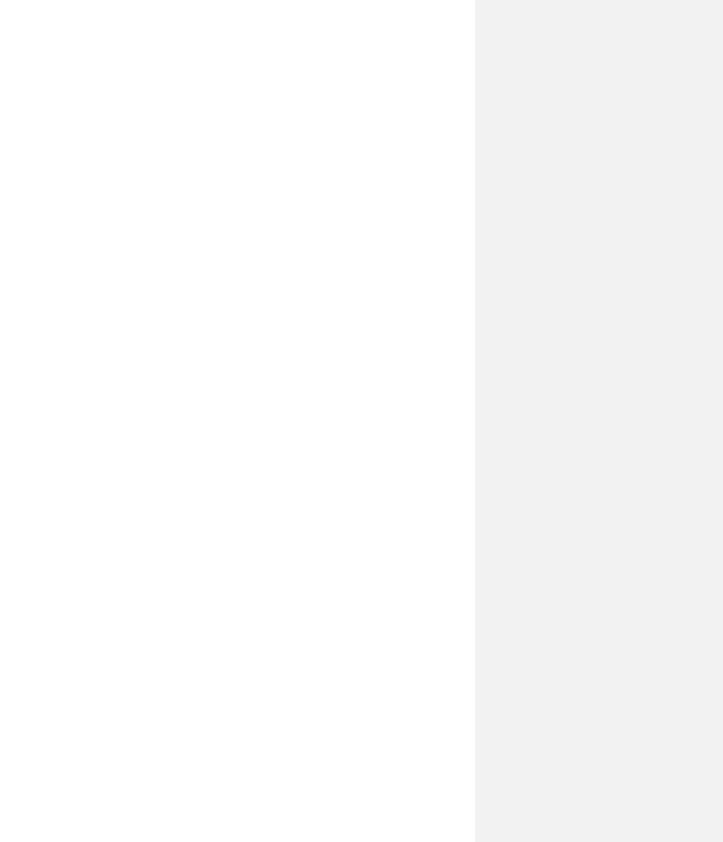
VCtot = EPower ∙ WG3 + Wcons ∙ CW + Cpower ∙ WG3 + EEn,tax ∙ Cpower |
(29) |
5.1.2.4 Break-even Spot Price
Break-even can be defined as the point in which the total cost is equal to the total income, resulting in a zero-profit scenario. Therefore, the objective of this section is to determine break-even spot price of the respective hydrogen price. All spot prices below the break-even spot price are considered to be profitable electricity prices for hydrogen production. This is accomplished by combining the two economic models (opportunity cost and contribution margin discussed in section 5.2) of the two alternatives as shown in equation (30). Thereafter, the break-even spot price can be computed.
Hydrogen price = (EPower + Cpower) ∙ (WG3) + (EEn,tax ∙ Cpower) + Wwater (30)
Furthermore, since the hydrogen price ranges from 3–7 €/kg as described in section 3.3.1, five different breakeven spot prices are obtained from equation (30). These prices are listed in Table 29–31. The target years have different break-even spot prices, and this is due to different prices of electricity certificate and energy fee, as seen in Table 23 24. Moreover, when the spot price approaches the break-even point, it will result in a decreased profitability of hydrogen production and vice versa.
Table 29. Breakeven Spot price as function of hydrogen selling price for year 2019.
Hydrogen Price |
Breakeven Electricity Cost |
|
|
|
|
|
|
|
3 [€/kg H2] |
49.3 |
[€/MWh] |
4 [€/kg H2] |
69.2 |
[€/MWh] |
5 [€/kg H2] |
89.13 |
[€/MWh] |
6 [€/kg H2] |
109.1 |
[€/MWh] |
7 [€/kg H2] |
129 |
[€/MWh] |
Table 30. Breakeven Spot price as function of hydrogen selling price for year 2020.
Hydrogen Price |
Breakeven Electricity Cost |
|
|
|
|
|
|
|
3 [€/kg H2] |
50.7 |
[€/MWh] |
4 [€/kg H2] |
70.7 |
[€/MWh] |
5 [€/kg H2] |
90.6 |
[€/MWh] |
6 [€/kg H2] |
110.5 |
[€/MWh] |
7 [€/kg H2] |
130.5 |
[€/MWh] |
|
|
|
55

Table 31. Breakeven Spot price as function of hydrogen selling price for year 2021.
Hydrogen Price |
Breakeven Electricity Cost |
|
|
|
|
|
|
|
3 [€/kg H2] |
52.3 |
[€/MWh] |
4 [€/kg H2] |
72.2 |
[€/MWh] |
5 [€/kg H2] |
92.2 |
[€/MWh] |
6 [€/kg H2] |
112.1 |
[€/MWh] |
7 [€/kg H2] |
132.1 |
[€/MWh] |
|
|
|
5.2 Economic Modeling Tools
This section will present two types of economic modelling tools, contribution margin and opportunity cost. The objective is to describe how these models are used to evaluate the respective alternatives. Given that this report aims to determine the spot prices at which production of becomes profitable, these two models are well-suited for such purpose.
5.2.1 Contribution Margin
The contribution margin serves a tool for companies to determine whether producing a specific product is profitable. Contribution margin can be calculated by subtracting the variable production cost of the product from the revenue generated through sales, as shown in equation (31). A positive resulting value signifies that a positive income (the revenue minus variable costs) is generated, while a negative value refers to a negative income [77][78].
Contribution margin = Total variable income − Total variable cost |
(31) |
5.2.2 Opportunity Cost
The contribution cost is the loss of a benefit when choosing a particular option over other potential options. This type of analysis is an economic method used to compare the economic resulting outcome of each option, with the aim of selecting the option that yields the most desirable economic outcome. Furthermore, in this report, the opportunity cost involves comparing the resulting outcome of the two alternatives.
5.2.3 Annuity Method
In this report, the annuity method is applied to determine the annual investment cost of the hydrogen production facility. This is obtained by multiplying respective facility’s total capital cost (for its whole lifespan) with an annuity constant. The annuity constant is based on the facility’s lifespan and a discount rate, which are listed in Table 32. The selected lifespan of 15 years is based on the average lifespan (10–20 years) of a proton exchange membrane electrolyzer [79]. The facilities annual fixed costs are meant to be covered by the annual incomes generated from sales of hydrogen, for the entire lifecycle of the facility.
56

Table 32. Parameters of Annuity Method [80].
Parameter |
Value |
|
|
|
|
Lifespan |
15 [years] |
Discount rate |
7 [%] |
Annuity constant |
0.110 |
|
|
57

CHAPTER 6. RESULTS
6.1 Simulations of Hydrogen Production
This chapter will present the resulting outcome of respective hydrogen facility, starting with analyzing the conducted simulations of the hydrogen productions taking place in the years 2019, 2020 and 2021. The simulations provide information about the quantity of hydrogen produced, which in turn was used to calculate the corresponding generated income. Thereafter, the profitability of respective facility is determined by subtracting the fixed capital investment from the variable income of that facility. Finally, the chapter will present within which range of the transition stage these facilities can contribute to the Gävle harbor’s transitioning process from diesel to hydrogen fuel.
6.1.1 Annual Hydrogen Production
Simulations of the annual hydrogen production of the three facilities with respect to the breakeven spot and hydrogen prices for each target year, are plotted and available in Appendix D as Figure 30–44. Based on these simulations, the amount of hydrogen produced as a function of operational hours of the electrolyzer, along with the corresponding income generated per target year could be obtained and are in detail listed in Table 40 42 for the 5 MW facility, Table 43 45 for the 10 MW facility and Table 46 48 for the 20 MW facility in Appendix B. The following Tables 33 35 are a summary of Table 40–48 in Appendix B, for all target years.
Table 33. Annual income generated for 5 MW, 10 MW and 20 MW facilities in year 2019, where hydrogen price ranges from 3–7 €/kg.
Spot Price |
Hydrogen Price |
Annual Income 5 MW |
Annual Income 10 MW |
Annual Income 20 MW |
|
||
|
|
|
|
|
|
|
|
|
|
|
|
|
|
|
|
49.3 |
[€/MWh] |
3 [€/kg] |
1.26 [m€] |
2.07 [m€] |
3.06 [m€] |
||
69.2 |
[€/MWh] |
4 [€/kg] |
1.97 [m€] |
3.27 [m€] |
4.87 [m€] |
||
89.13 |
[€/MWh] |
5 [€/kg] |
2.47 [m€] |
4.11 [m€] |
6.11 [m€] |
||
109.1 |
[€/MWh] |
6 [€/kg] |
2.97 [m€] |
4.94 [m€] |
7.33 [m€] |
||
129 |
[€/MWh] |
7 [€/kg] |
3.46 [m€] |
5.76 [m€] |
8.56 [€m] |
||
|
|
|
|
|
|
|
|
Table 34. Annual income generated for 5 MW, 10 MW and 20 MW in year 2020, where hydrogen price ranges from 3–7 €/kg.
Spot Price |
Hydrogen Price |
Annual Income 5 MW |
Annual Income 10 MW |
Annual Income 20 MW |
|
||
|
|
|
|
|
|
|
|
|
|
|
|
|
|
|
|
50.7 |
[€/MWh] |
3 [€/kg] |
1.52 [m€] |
2.47 [m€] |
3.56 [m€] |
||
70.7 |
[€/MWh] |
4 [€/kg] |
2.04 [m€] |
3.32 [m€] |
4.78 [m€] |
||
90.6 |
[€/MWh] |
5 [€/kg] |
2.56 [m€] |
4.15 [m€] |
5.98 [m€] |
||
110.5 |
[€/MWh] |
6 [€/kg] |
3.07 [m€] |
4.98 [m€] |
7.18 [m€] |
||
130.5 |
[€/MWh] |
7 [€/kg] |
3.58 [m€] |
5.81 [m€] |
8.37 [m€] |
||
|
|
|
|
|
|
|
|
Table 35. Annual income generated for 5 MW, 10 MW and 20 MW in year 2021, where hydrogen price ranges from 3–7 €/kg.
Spot Price |
Hydrogen Price |
Annual Income 5 MW |
Annual Income 10 MW |
Annual Income 20 MW |
|
||
|
|
|
|
|
|
|
|
|
|
|
|
|
|
|
|
52.3 |
[€/MWh] |
3 [€/kg] |
1.26 [m€] |
2.13 [m€] |
3.16 [m€] |
||
72.2 |
[€/MWh] |
4 [€/kg] |
2.10 [m€] |
3.51 [m€] |
5.18 [m€] |
||
92.2 |
[€/MWh] |
5 [€/kg] |
2.69 [m€] |
4.50 [m€] |
6.62 [m€] |
||
112.1 |
[€/MWh] |
6 [€/kg] |
3.27 [m€] |
5.47 [m€] |
8.10 [m€] |
||
132.1 |
[€/MWh] |
7 [€/kg] |
3.84 [m€] |
6.42 [m€] |
9.43 [m€] |
||
|
|
|
|
|
|
|
|
58