
- •Preface
- •Imaging Microscopic Features
- •Measuring the Crystal Structure
- •References
- •Contents
- •1.4 Simulating the Effects of Elastic Scattering: Monte Carlo Calculations
- •What Are the Main Features of the Beam Electron Interaction Volume?
- •How Does the Interaction Volume Change with Composition?
- •How Does the Interaction Volume Change with Incident Beam Energy?
- •How Does the Interaction Volume Change with Specimen Tilt?
- •1.5 A Range Equation To Estimate the Size of the Interaction Volume
- •References
- •2: Backscattered Electrons
- •2.1 Origin
- •2.2.1 BSE Response to Specimen Composition (η vs. Atomic Number, Z)
- •SEM Image Contrast with BSE: “Atomic Number Contrast”
- •SEM Image Contrast: “BSE Topographic Contrast—Number Effects”
- •2.2.3 Angular Distribution of Backscattering
- •Beam Incident at an Acute Angle to the Specimen Surface (Specimen Tilt > 0°)
- •SEM Image Contrast: “BSE Topographic Contrast—Trajectory Effects”
- •2.2.4 Spatial Distribution of Backscattering
- •Depth Distribution of Backscattering
- •Radial Distribution of Backscattered Electrons
- •2.3 Summary
- •References
- •3: Secondary Electrons
- •3.1 Origin
- •3.2 Energy Distribution
- •3.3 Escape Depth of Secondary Electrons
- •3.8 Spatial Characteristics of Secondary Electrons
- •References
- •4: X-Rays
- •4.1 Overview
- •4.2 Characteristic X-Rays
- •4.2.1 Origin
- •4.2.2 Fluorescence Yield
- •4.2.3 X-Ray Families
- •4.2.4 X-Ray Nomenclature
- •4.2.6 Characteristic X-Ray Intensity
- •Isolated Atoms
- •X-Ray Production in Thin Foils
- •X-Ray Intensity Emitted from Thick, Solid Specimens
- •4.3 X-Ray Continuum (bremsstrahlung)
- •4.3.1 X-Ray Continuum Intensity
- •4.3.3 Range of X-ray Production
- •4.4 X-Ray Absorption
- •4.5 X-Ray Fluorescence
- •References
- •5.1 Electron Beam Parameters
- •5.2 Electron Optical Parameters
- •5.2.1 Beam Energy
- •Landing Energy
- •5.2.2 Beam Diameter
- •5.2.3 Beam Current
- •5.2.4 Beam Current Density
- •5.2.5 Beam Convergence Angle, α
- •5.2.6 Beam Solid Angle
- •5.2.7 Electron Optical Brightness, β
- •Brightness Equation
- •5.2.8 Focus
- •Astigmatism
- •5.3 SEM Imaging Modes
- •5.3.1 High Depth-of-Field Mode
- •5.3.2 High-Current Mode
- •5.3.3 Resolution Mode
- •5.3.4 Low-Voltage Mode
- •5.4 Electron Detectors
- •5.4.1 Important Properties of BSE and SE for Detector Design and Operation
- •Abundance
- •Angular Distribution
- •Kinetic Energy Response
- •5.4.2 Detector Characteristics
- •Angular Measures for Electron Detectors
- •Elevation (Take-Off) Angle, ψ, and Azimuthal Angle, ζ
- •Solid Angle, Ω
- •Energy Response
- •Bandwidth
- •5.4.3 Common Types of Electron Detectors
- •Backscattered Electrons
- •Passive Detectors
- •Scintillation Detectors
- •Semiconductor BSE Detectors
- •5.4.4 Secondary Electron Detectors
- •Everhart–Thornley Detector
- •Through-the-Lens (TTL) Electron Detectors
- •TTL SE Detector
- •TTL BSE Detector
- •Measuring the DQE: BSE Semiconductor Detector
- •References
- •6: Image Formation
- •6.1 Image Construction by Scanning Action
- •6.2 Magnification
- •6.3 Making Dimensional Measurements With the SEM: How Big Is That Feature?
- •Using a Calibrated Structure in ImageJ-Fiji
- •6.4 Image Defects
- •6.4.1 Projection Distortion (Foreshortening)
- •6.4.2 Image Defocusing (Blurring)
- •6.5 Making Measurements on Surfaces With Arbitrary Topography: Stereomicroscopy
- •6.5.1 Qualitative Stereomicroscopy
- •Fixed beam, Specimen Position Altered
- •Fixed Specimen, Beam Incidence Angle Changed
- •6.5.2 Quantitative Stereomicroscopy
- •Measuring a Simple Vertical Displacement
- •References
- •7: SEM Image Interpretation
- •7.1 Information in SEM Images
- •7.2.2 Calculating Atomic Number Contrast
- •Establishing a Robust Light-Optical Analogy
- •Getting It Wrong: Breaking the Light-Optical Analogy of the Everhart–Thornley (Positive Bias) Detector
- •Deconstructing the SEM/E–T Image of Topography
- •SUM Mode (A + B)
- •DIFFERENCE Mode (A−B)
- •References
- •References
- •9: Image Defects
- •9.1 Charging
- •9.1.1 What Is Specimen Charging?
- •9.1.3 Techniques to Control Charging Artifacts (High Vacuum Instruments)
- •Observing Uncoated Specimens
- •Coating an Insulating Specimen for Charge Dissipation
- •Choosing the Coating for Imaging Morphology
- •9.2 Radiation Damage
- •9.3 Contamination
- •References
- •10: High Resolution Imaging
- •10.2 Instrumentation Considerations
- •10.4.1 SE Range Effects Produce Bright Edges (Isolated Edges)
- •10.4.4 Too Much of a Good Thing: The Bright Edge Effect Hinders Locating the True Position of an Edge for Critical Dimension Metrology
- •10.5.1 Beam Energy Strategies
- •Low Beam Energy Strategy
- •High Beam Energy Strategy
- •Making More SE1: Apply a Thin High-δ Metal Coating
- •Making Fewer BSEs, SE2, and SE3 by Eliminating Bulk Scattering From the Substrate
- •10.6 Factors That Hinder Achieving High Resolution
- •10.6.2 Pathological Specimen Behavior
- •Contamination
- •Instabilities
- •References
- •11: Low Beam Energy SEM
- •11.3 Selecting the Beam Energy to Control the Spatial Sampling of Imaging Signals
- •11.3.1 Low Beam Energy for High Lateral Resolution SEM
- •11.3.2 Low Beam Energy for High Depth Resolution SEM
- •11.3.3 Extremely Low Beam Energy Imaging
- •References
- •12.1.1 Stable Electron Source Operation
- •12.1.2 Maintaining Beam Integrity
- •12.1.4 Minimizing Contamination
- •12.3.1 Control of Specimen Charging
- •12.5 VPSEM Image Resolution
- •References
- •13: ImageJ and Fiji
- •13.1 The ImageJ Universe
- •13.2 Fiji
- •13.3 Plugins
- •13.4 Where to Learn More
- •References
- •14: SEM Imaging Checklist
- •14.1.1 Conducting or Semiconducting Specimens
- •14.1.2 Insulating Specimens
- •14.2 Electron Signals Available
- •14.2.1 Beam Electron Range
- •14.2.2 Backscattered Electrons
- •14.2.3 Secondary Electrons
- •14.3 Selecting the Electron Detector
- •14.3.2 Backscattered Electron Detectors
- •14.3.3 “Through-the-Lens” Detectors
- •14.4 Selecting the Beam Energy for SEM Imaging
- •14.4.4 High Resolution SEM Imaging
- •Strategy 1
- •Strategy 2
- •14.5 Selecting the Beam Current
- •14.5.1 High Resolution Imaging
- •14.5.2 Low Contrast Features Require High Beam Current and/or Long Frame Time to Establish Visibility
- •14.6 Image Presentation
- •14.6.1 “Live” Display Adjustments
- •14.6.2 Post-Collection Processing
- •14.7 Image Interpretation
- •14.7.1 Observer’s Point of View
- •14.7.3 Contrast Encoding
- •14.8.1 VPSEM Advantages
- •14.8.2 VPSEM Disadvantages
- •15: SEM Case Studies
- •15.1 Case Study: How High Is That Feature Relative to Another?
- •15.2 Revealing Shallow Surface Relief
- •16.1.2 Minor Artifacts: The Si-Escape Peak
- •16.1.3 Minor Artifacts: Coincidence Peaks
- •16.1.4 Minor Artifacts: Si Absorption Edge and Si Internal Fluorescence Peak
- •16.2 “Best Practices” for Electron-Excited EDS Operation
- •16.2.1 Operation of the EDS System
- •Choosing the EDS Time Constant (Resolution and Throughput)
- •Choosing the Solid Angle of the EDS
- •Selecting a Beam Current for an Acceptable Level of System Dead-Time
- •16.3.1 Detector Geometry
- •16.3.2 Process Time
- •16.3.3 Optimal Working Distance
- •16.3.4 Detector Orientation
- •16.3.5 Count Rate Linearity
- •16.3.6 Energy Calibration Linearity
- •16.3.7 Other Items
- •16.3.8 Setting Up a Quality Control Program
- •Using the QC Tools Within DTSA-II
- •Creating a QC Project
- •Linearity of Output Count Rate with Live-Time Dose
- •Resolution and Peak Position Stability with Count Rate
- •Solid Angle for Low X-ray Flux
- •Maximizing Throughput at Moderate Resolution
- •References
- •17: DTSA-II EDS Software
- •17.1 Getting Started With NIST DTSA-II
- •17.1.1 Motivation
- •17.1.2 Platform
- •17.1.3 Overview
- •17.1.4 Design
- •Simulation
- •Quantification
- •Experiment Design
- •Modeled Detectors (. Fig. 17.1)
- •Window Type (. Fig. 17.2)
- •The Optimal Working Distance (. Figs. 17.3 and 17.4)
- •Elevation Angle
- •Sample-to-Detector Distance
- •Detector Area
- •Crystal Thickness
- •Number of Channels, Energy Scale, and Zero Offset
- •Resolution at Mn Kα (Approximate)
- •Azimuthal Angle
- •Gold Layer, Aluminum Layer, Nickel Layer
- •Dead Layer
- •Zero Strobe Discriminator (. Figs. 17.7 and 17.8)
- •Material Editor Dialog (. Figs. 17.9, 17.10, 17.11, 17.12, 17.13, and 17.14)
- •17.2.1 Introduction
- •17.2.2 Monte Carlo Simulation
- •17.2.4 Optional Tables
- •References
- •18: Qualitative Elemental Analysis by Energy Dispersive X-Ray Spectrometry
- •18.1 Quality Assurance Issues for Qualitative Analysis: EDS Calibration
- •18.2 Principles of Qualitative EDS Analysis
- •Exciting Characteristic X-Rays
- •Fluorescence Yield
- •X-ray Absorption
- •Si Escape Peak
- •Coincidence Peaks
- •18.3 Performing Manual Qualitative Analysis
- •Beam Energy
- •Choosing the EDS Resolution (Detector Time Constant)
- •Obtaining Adequate Counts
- •18.4.1 Employ the Available Software Tools
- •18.4.3 Lower Photon Energy Region
- •18.4.5 Checking Your Work
- •18.5 A Worked Example of Manual Peak Identification
- •References
- •19.1 What Is a k-ratio?
- •19.3 Sets of k-ratios
- •19.5 The Analytical Total
- •19.6 Normalization
- •19.7.1 Oxygen by Assumed Stoichiometry
- •19.7.3 Element by Difference
- •19.8 Ways of Reporting Composition
- •19.8.1 Mass Fraction
- •19.8.2 Atomic Fraction
- •19.8.3 Stoichiometry
- •19.8.4 Oxide Fractions
- •Example Calculations
- •19.9 The Accuracy of Quantitative Electron-Excited X-ray Microanalysis
- •19.9.1 Standards-Based k-ratio Protocol
- •19.9.2 “Standardless Analysis”
- •19.10 Appendix
- •19.10.1 The Need for Matrix Corrections To Achieve Quantitative Analysis
- •19.10.2 The Physical Origin of Matrix Effects
- •19.10.3 ZAF Factors in Microanalysis
- •X-ray Generation With Depth, φ(ρz)
- •X-ray Absorption Effect, A
- •X-ray Fluorescence, F
- •References
- •20.2 Instrumentation Requirements
- •20.2.1 Choosing the EDS Parameters
- •EDS Spectrum Channel Energy Width and Spectrum Energy Span
- •EDS Time Constant (Resolution and Throughput)
- •EDS Calibration
- •EDS Solid Angle
- •20.2.2 Choosing the Beam Energy, E0
- •20.2.3 Measuring the Beam Current
- •20.2.4 Choosing the Beam Current
- •Optimizing Analysis Strategy
- •20.3.4 Ba-Ti Interference in BaTiSi3O9
- •20.4 The Need for an Iterative Qualitative and Quantitative Analysis Strategy
- •20.4.2 Analysis of a Stainless Steel
- •20.5 Is the Specimen Homogeneous?
- •20.6 Beam-Sensitive Specimens
- •20.6.1 Alkali Element Migration
- •20.6.2 Materials Subject to Mass Loss During Electron Bombardment—the Marshall-Hall Method
- •Thin Section Analysis
- •Bulk Biological and Organic Specimens
- •References
- •21: Trace Analysis by SEM/EDS
- •21.1 Limits of Detection for SEM/EDS Microanalysis
- •21.2.1 Estimating CDL from a Trace or Minor Constituent from Measuring a Known Standard
- •21.2.2 Estimating CDL After Determination of a Minor or Trace Constituent with Severe Peak Interference from a Major Constituent
- •21.3 Measurements of Trace Constituents by Electron-Excited Energy Dispersive X-ray Spectrometry
- •The Inevitable Physics of Remote Excitation Within the Specimen: Secondary Fluorescence Beyond the Electron Interaction Volume
- •Simulation of Long-Range Secondary X-ray Fluorescence
- •NIST DTSA II Simulation: Vertical Interface Between Two Regions of Different Composition in a Flat Bulk Target
- •NIST DTSA II Simulation: Cubic Particle Embedded in a Bulk Matrix
- •21.5 Summary
- •References
- •22.1.2 Low Beam Energy Analysis Range
- •22.2 Advantage of Low Beam Energy X-Ray Microanalysis
- •22.2.1 Improved Spatial Resolution
- •22.3 Challenges and Limitations of Low Beam Energy X-Ray Microanalysis
- •22.3.1 Reduced Access to Elements
- •22.3.3 At Low Beam Energy, Almost Everything Is Found To Be Layered
- •Analysis of Surface Contamination
- •References
- •23: Analysis of Specimens with Special Geometry: Irregular Bulk Objects and Particles
- •23.2.1 No Chemical Etching
- •23.3 Consequences of Attempting Analysis of Bulk Materials With Rough Surfaces
- •23.4.1 The Raw Analytical Total
- •23.4.2 The Shape of the EDS Spectrum
- •23.5 Best Practices for Analysis of Rough Bulk Samples
- •23.6 Particle Analysis
- •Particle Sample Preparation: Bulk Substrate
- •The Importance of Beam Placement
- •Overscanning
- •“Particle Mass Effect”
- •“Particle Absorption Effect”
- •The Analytical Total Reveals the Impact of Particle Effects
- •Does Overscanning Help?
- •23.6.6 Peak-to-Background (P/B) Method
- •Specimen Geometry Severely Affects the k-ratio, but Not the P/B
- •Using the P/B Correspondence
- •23.7 Summary
- •References
- •24: Compositional Mapping
- •24.2 X-Ray Spectrum Imaging
- •24.2.1 Utilizing XSI Datacubes
- •24.2.2 Derived Spectra
- •SUM Spectrum
- •MAXIMUM PIXEL Spectrum
- •24.3 Quantitative Compositional Mapping
- •24.4 Strategy for XSI Elemental Mapping Data Collection
- •24.4.1 Choosing the EDS Dead-Time
- •24.4.2 Choosing the Pixel Density
- •24.4.3 Choosing the Pixel Dwell Time
- •“Flash Mapping”
- •High Count Mapping
- •References
- •25.1 Gas Scattering Effects in the VPSEM
- •25.1.1 Why Doesn’t the EDS Collimator Exclude the Remote Skirt X-Rays?
- •25.2 What Can Be Done To Minimize gas Scattering in VPSEM?
- •25.2.2 Favorable Sample Characteristics
- •Particle Analysis
- •25.2.3 Unfavorable Sample Characteristics
- •References
- •26.1 Instrumentation
- •26.1.2 EDS Detector
- •26.1.3 Probe Current Measurement Device
- •Direct Measurement: Using a Faraday Cup and Picoammeter
- •A Faraday Cup
- •Electrically Isolated Stage
- •Indirect Measurement: Using a Calibration Spectrum
- •26.1.4 Conductive Coating
- •26.2 Sample Preparation
- •26.2.1 Standard Materials
- •26.2.2 Peak Reference Materials
- •26.3 Initial Set-Up
- •26.3.1 Calibrating the EDS Detector
- •Selecting a Pulse Process Time Constant
- •Energy Calibration
- •Quality Control
- •Sample Orientation
- •Detector Position
- •Probe Current
- •26.4 Collecting Data
- •26.4.1 Exploratory Spectrum
- •26.4.2 Experiment Optimization
- •26.4.3 Selecting Standards
- •26.4.4 Reference Spectra
- •26.4.5 Collecting Standards
- •26.4.6 Collecting Peak-Fitting References
- •26.5 Data Analysis
- •26.5.2 Quantification
- •26.6 Quality Check
- •Reference
- •27.2 Case Study: Aluminum Wire Failures in Residential Wiring
- •References
- •28: Cathodoluminescence
- •28.1 Origin
- •28.2 Measuring Cathodoluminescence
- •28.3 Applications of CL
- •28.3.1 Geology
- •Carbonado Diamond
- •Ancient Impact Zircons
- •28.3.2 Materials Science
- •Semiconductors
- •Lead-Acid Battery Plate Reactions
- •28.3.3 Organic Compounds
- •References
- •29.1.1 Single Crystals
- •29.1.2 Polycrystalline Materials
- •29.1.3 Conditions for Detecting Electron Channeling Contrast
- •Specimen Preparation
- •Instrument Conditions
- •29.2.1 Origin of EBSD Patterns
- •29.2.2 Cameras for EBSD Pattern Detection
- •29.2.3 EBSD Spatial Resolution
- •29.2.5 Steps in Typical EBSD Measurements
- •Sample Preparation for EBSD
- •Align Sample in the SEM
- •Check for EBSD Patterns
- •Adjust SEM and Select EBSD Map Parameters
- •Run the Automated Map
- •29.2.6 Display of the Acquired Data
- •29.2.7 Other Map Components
- •29.2.10 Application Example
- •Application of EBSD To Understand Meteorite Formation
- •29.2.11 Summary
- •Specimen Considerations
- •EBSD Detector
- •Selection of Candidate Crystallographic Phases
- •Microscope Operating Conditions and Pattern Optimization
- •Selection of EBSD Acquisition Parameters
- •Collect the Orientation Map
- •References
- •30.1 Introduction
- •30.2 Ion–Solid Interactions
- •30.3 Focused Ion Beam Systems
- •30.5 Preparation of Samples for SEM
- •30.5.1 Cross-Section Preparation
- •30.5.2 FIB Sample Preparation for 3D Techniques and Imaging
- •30.6 Summary
- •References
- •31: Ion Beam Microscopy
- •31.1 What Is So Useful About Ions?
- •31.2 Generating Ion Beams
- •31.3 Signal Generation in the HIM
- •31.5 Patterning with Ion Beams
- •31.7 Chemical Microanalysis with Ion Beams
- •References
- •Appendix
- •A Database of Electron–Solid Interactions
- •A Database of Electron–Solid Interactions
- •Introduction
- •Backscattered Electrons
- •Secondary Yields
- •Stopping Powers
- •X-ray Ionization Cross Sections
- •Conclusions
- •References
- •Index
- •Reference List
- •Index

381 |
|
23 |
|
|
|
Analysis of Specimens with Special Geometry: Irregular Bulk Objects and Particles
23.1\ The Origins of “Geometric Effects”: Bulk Specimens – 382
23.2\ What Degree of Surface Finish Is Required for Electron-Excited X-ray Microanalysis To Minimize Geometric Effects? – 384
23.2.1\ No Chemical Etching – 384
23.3\ Consequences of Attempting Analysis of
Bulk Materials With Rough Surfaces – 385
23.4\ Useful Indicators of Geometric Factors Impact on Analysis – 386
23.4.1\ The Raw Analytical Total – 386 23.4.2\ The Shape of the EDS Spectrum – 389
23.5\ Best Practices for Analysis of Rough Bulk Samples – 391
23.6\ Particle Analysis – 394
23.6.1\ How Do X-ray Measurements of Particles Differ From Bulk Measurements? – 394
23.6.2\ Collecting Optimum Spectra From Particles – 395 23.6.3\ X-ray Spectrum Imaging: Understanding Heterogeneous
Materials – 400
23.6.4\ Particle Geometry Factors Influencing Quantitative Analysis of Particles – 403
23.6.5\ Uncertainty in Quantitative Analysis of Particles – 405 23.6.6\ Peak-to-Background (P/B) Method – 408
23.7\ Summary – 410
\References – 411
© Springer Science+Business Media LLC 2018
J. Goldstein et al., Scanning Electron Microscopy and X-Ray Microanalysis, https://doi.org/10.1007/978-1-4939-6676-9_23

\382 Chapter 23 · Analysis of Specimens with Special Geometry: Irregular Bulk Objects and Particles
There are two “zero-th level” assumptions that underpin the basis for quantitative electron-excited X-ray microanalysis: \1.\ The only reason that the measured X-ray intensity differs between the unknown and the standard(s) is that the composition is different. No other factors such as the
specimen shape, orientation, or size influence the measured X-ray spectrum.
\2.\ The specimen is homogeneous in composition over the volume excited by the electron beam from which the characteristic and continuum X-rays are emitted, including the secondary radiation induced by absorption of the primary electron-excited radiation.
When either of these conditions is not met, a significant increase in the overall uncertainty budget of the analysis can occur beyond the ideal situation in which the uncertainties arise from counting statistics and from uncertainties in the calculated matrix correction factors.
Considering “zeroth-level” assumption 1, sample geometry can significantly modify the measured X-ray intensity. The ideal specimen is flat and placed at known angles to the incident beam and the X-ray detector(s). Topographic features on bulk specimens (defined as those for which the thickness is much greater than the electron range) or unusual geometric shapes, such as particles with dimensions similar to the electron range, can strongly affect the measured X-rays by modifying X-ray generation and by affecting the loss of X-rays due to absorption. In severe cases, the impact of “geometric factors” on the final concentrations becomes so large as to render the compositional results, as calculated with the conventional standards-based/matrix corrections protocol or the standardless protocol, nearly worthless.
Cu E0 = 20 keV
|
0.8 |
|
coefficient |
0.7 |
|
0.6 |
||
|
||
Backscatter |
0.5 |
|
0.4 |
||
|
||
|
0.3 |
|
|
0.2 |
0 |
20 |
40 |
60 |
80 |
|
|
Tilt angle (degrees) |
|
. Fig. 23.1 Backscatter coefficient η vs. surface tilt θ (inclination) for Cu at E0 = 20 keV as calculated with the Monte Carlo electron trajectory simulation embedded in NIST DTSA-II
is at a minimum, BSEs carry off energy which would have gone to cause additional inner shell ionization events followed by subsequent X-ray emission had those electrons remained in the specimen. As the local surface inclination (tilt) increases, backscattering increases and more X-ray generation is lost compared to normal beam incidence situation.
. Figure 23.2 shows Monte Carlo calculations of the Cu K-L2,3 X-ray intensity emitted from a flat, bulk copper specimen, expressed as a “k-ratio,” where the denominator is the intensity emitted from copper at zero tilt (normal beam incidence). As the local surface inclination (tilt angle) increases above zero degrees, the X-ray production decreases with
23.1\ The Origins of “Geometric Effects”:
Bulk Specimens
The ideal sample is compositionally homogeneous on a microscopic scale, has a flat surface, and is set at known angles to the incident electron beam and the X-ray spectrometer. Compared to the X-ray spectrum measured from this ideal spectrum, geometric effects occur when the size and shape of the specimen (1) modify the interaction of the electrons with the specimen so as to affect the generated X-ray intensity and (2) alter the length of the absorption path along which the generated X-rays travel to escape the specimen and reach the detector so as to affect the measured X-ray intensity.
Because electron backscattering depends on the local surface inclination to the incident beam, tilted samples generate fewer X-rays compared to a sample at normal beam incidence (0° tilt). An illustration of this effect for bulk copper, as
23 calculated with the Monte Carlo simulation embedded in NIST DTSA-II, is shown in . Fig. 23.1. Even at normal beam incidence where the backscattered electron (BSE) coefficient
Cu K-L2,3 emission vs. Tilt (E0 = 20 keV)
|
1.0 |
|
ratio-k |
0.8 |
|
0.6 |
||
|
||
|
0.4 |
0.2
0.0
0 |
20 |
40 |
60 |
80 |
|
|
Tilt angle (degrees) |
|
. Fig. 23.2 Emitted Cu K-L2,3 X-ray intensity calculated as the k-ratio relative to the intensity at a tilt of 0°, vs. surface tilt θ (inclination), for Cu at E0 = 20 keV as calculated with the Monte Carlo electron trajectory simulation embedded in NIST DTSA-II

23.1 · The Origins of “Geometric Effects”: Bulk Specimens
. Fig. 23.3 Schematic illustration of the effects of surface topography on the X-ray absorption path length within the specimen
Normal flat bulk target absorption path
Standard
383 |
|
23 |
|
|
|
Geometric Effects: surface roughness affects local absorption path to reach detector
I/I0 = exp[-(µ/ρ)ρs]
ψ
|
|
Depth |
|
|
|
of surface |
|
|
Reduced |
scratch |
|
Extended |
|
||
|
|||
absorption |
Extended |
||
absorption |
|||
path due to |
|||
path due to |
absorption |
||
scratch |
|||
ridge |
path due to |
||
|
scratch
increasing tilt due to the increased backscattering seen in
. Fig. 23.1. There is a relatively small decrease in the k-ratio at low tilt angles, but the k-ratio decreases rapidly for tilt angles above approximately 40°. Because of the high photon energy of Cu K-L2,3 (8.04 keV) and the relative transparency of any material to its own X-rays, there is no significant absorption so that the behavior shown in . Fig. 23.2 is almost entirely due to the modification of the production of X-rays due to backscatter loss.
Surface topography modifies the X-ray absorption path length to the detector compared to a flat specimen at normal beam incidence. As shown schematically in . Fig. 23.3, topographic features such as scratches and ridges can increase or decrease the absorption path length in the direction of the X-ray detector. X-ray absorption follows an exponential dependence on this path length:
|
0 |
( |
|
) |
|
|
|
I / I |
|
= exp − |
µ / ρ |
|
ρs |
\ |
(23.1) |
|
|
|
|
|
|
|
where I0 is the initial intensity, I is the intensity that remains after passing through a path length s (cm), (μ/ρ) is the mass absorption coefficient (cm2/g) for the photon energy of interest that depends on the absorption contributions of all elements present, and ρ is the density (g/cm3). Considering the entire energy range of the generated X-ray spectrum, which extends from a practical minimum threshold of 100 eV to the incident beam energy, E0 (the Duane–Hunt limit), as the X-ray photon energy decreases, absorption generally increases. Absorption is especially strong if the photon energy is less than 1 keV above the critical ionization energy for any elemental constituent in the specimen. An example of this effect is shown in . Fig. 23.4
|
|
|
X-ray absorption |
|
|
|
|||
1.0 |
|
|
|
|
|
|
|
|
|
|
|
|
|
|
|
|
|
||
|
|
|
|
|
|
|
|
|
|
0.8 |
|
|
|
|
|
Al K-L2,3 in Si |
|
|
|
|
|
|
|
|
|
|
|||
0.6 |
|
|
|
|
|
Si K-L2,3 in AI |
|
|
|
|
|
|
|
|
|
|
|||
|
|
|
|
|
|
|
|
|
|
0 |
|
|
|
|
|
|
|
|
|
I/I |
|
|
|
|
|
|
|
|
|
0.4 |
|
|
|
|
|
|
|
|
|
0.2 |
|
|
|
|
|
|
|
|
|
0.0 |
|
|
|
|
|
|
|
|
|
|
|
|
|
|
|
|
|
|
|
0 |
2 |
4 |
|
6 |
8 |
10 |
Absorption path length (mm)
. Fig. 23.4 Absorption as a function of path length for Al K-L2,3
(1.487 keV) passing through Si (Kcrit = 1.838 keV), and Si K-L2,3 (1.740 keV) passing through Al (Kcrit = 1.559 keV)
for absorption as a function of path length for two contrasting
cases:AlK-L2,3 (1.487keV)passingthroughSi(Kcrit =1.838keV), and Si K-L2,3 (1.740 keV) passing through Al (Kcrit =1.559 keV). Because Si K-L2,3 is 0.181 keV above the critical ionization
energyforAl,SiisverystronglyabsorbedbyAl(μ/ρ=3282cm2/g) such that there is no penetration beyond approximately 6 μm. By comparison, Al K-L2,3 is below the critical ionization energy for Si, so it much less strongly absorbed (μ/ρ=535 cm2/g), with approximately 50% of Al K-L2,3 intensity still remaining after 6-μm penetration through Si.
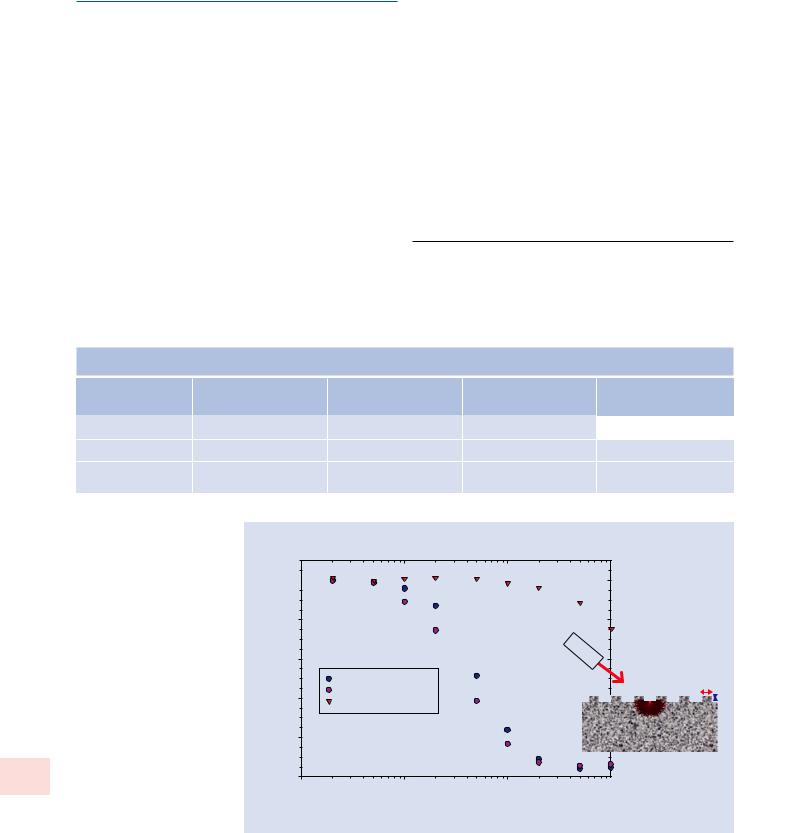
\384 Chapter 23 · Analysis of Specimens with Special Geometry: Irregular Bulk Objects and Particles
23.2\ What Degree of Surface Finish Is
Required for Electron-Excited X-ray
Microanalysis To Minimize Geometric
Effects?
Early in the history of microanalysis by electron-excited X-ray spectrometry, it was recognized that controlling the surface condition of a specimen was critical to achieving high-accu- racy results by reducing geometric effects to a negligible level. Yakowitz and Heinrich (1968) performed a series of experiments in which the metallographic preparation sequence of grinding and polishing was interrupted at various stages. Materials examined included pure elements with two widely different characteristic peak energies, for example, Au M5-
N6,7 at 2.123 keV and Au L3-M4,5 at 9.711 keV, and homogeneous binary metal alloys with widely differing characteristic
X-ray energies. For each surface condition, the characteristic
X-ray intensity was then measured at random locations and along line traverses on the specimen surface to examine the variation in characteristic X-ray intensity that could be ascribed to surface roughness. Results for selected surface conditions for a gold target are listed in . Table 23.1. For the Au L3-M4,5 measurements, a final polish of the surface with 0.5-μm alumina was necessary to reduce the coefficient of
variation for 20 random measurements to a level similar to the expected variation from the random counting statistics,
expressed as 3 n1/2/n. For the lower photon energy Au M5-N6,7 which suffers stronger absorption, it was necessary to improve
the surface polish to 0.1 μm alumina to achieve similar results. For even lower photon energy peaks, such as those associated with low atomic number elements with Z ≤ 9 (fluorine) for which E < 1 keV, even better surface finish is required to control the geometric effects. Newbury and Ritchie (2013a) simulated X-ray emission from crenelated surfaces with the Monte Carlo simulation embedded in NIST DTSA-II to examine the influence of surface topography on low photon energy peaks. As shown in . Fig. 23.5 for FeO at an incident beam energy E0 = 10 keV, the depth of scratches had to be reduced below 50 nm to reduce the geometric effects on O K,
Fe L3-M4,5, and Fe K-L2,3 to a negligible level.
23.2.1\ No Chemical Etching
In addition to achieving a high degree of surface finish to minimize geometric effects, it is also important to avoid chemical or electrochemical etching of the final surface. For effective optical microscopy of microstructures, chemical
. Table 23.1 Characteristic X-ray intensity measured on gold after various stages of grinding and polishing (Yakowitz and Heinrich 1968)
Surface condition |
AuMα (2.123 keV) |
|
Coeff. variation,% |
600 grit SiC |
8.6 |
0.5 μm Al2O3 |
0.7 |
0.1 μm Al2O3 |
0.46 |
. Fig. 23.5 Plots of O K, Fe L3-
M4,5, and Fe K-L2,3 as a function of scratch depth for a crenelated
surface as calculated with the
Monte Carlo simulation embed- 1.0 ded in NIST DTSA-II (Newbury
and Ritchie 2013a,b)
|
|
0.8 |
|
ratio-k |
0.6 |
|
|
|
|
|
0.4 |
|
|
0.2 |
23 |
|
0.0 |
0.01
AuMα (2.123 keV) |
AuLα (9.711 keV) |
AuLα (9.711 keV) |
3 n1/2/n,% |
Coeff. variation,% |
n1/2/n,% |
0.39 |
1.8 |
0.93 |
|
|
|
0.39 |
1.1 |
0.93 |
0.39 |
0.42 |
0.93 |
FeO E0 = 10 keV
EDS
O K-L2,3 (0.523 keV)
Fe L (0.704 keV)
FeK-L2,3 (6.400 keV)
0.1 |
1 |
10 |
Scratch depth and period (micrometers)