
- •Preface
- •Imaging Microscopic Features
- •Measuring the Crystal Structure
- •References
- •Contents
- •1.4 Simulating the Effects of Elastic Scattering: Monte Carlo Calculations
- •What Are the Main Features of the Beam Electron Interaction Volume?
- •How Does the Interaction Volume Change with Composition?
- •How Does the Interaction Volume Change with Incident Beam Energy?
- •How Does the Interaction Volume Change with Specimen Tilt?
- •1.5 A Range Equation To Estimate the Size of the Interaction Volume
- •References
- •2: Backscattered Electrons
- •2.1 Origin
- •2.2.1 BSE Response to Specimen Composition (η vs. Atomic Number, Z)
- •SEM Image Contrast with BSE: “Atomic Number Contrast”
- •SEM Image Contrast: “BSE Topographic Contrast—Number Effects”
- •2.2.3 Angular Distribution of Backscattering
- •Beam Incident at an Acute Angle to the Specimen Surface (Specimen Tilt > 0°)
- •SEM Image Contrast: “BSE Topographic Contrast—Trajectory Effects”
- •2.2.4 Spatial Distribution of Backscattering
- •Depth Distribution of Backscattering
- •Radial Distribution of Backscattered Electrons
- •2.3 Summary
- •References
- •3: Secondary Electrons
- •3.1 Origin
- •3.2 Energy Distribution
- •3.3 Escape Depth of Secondary Electrons
- •3.8 Spatial Characteristics of Secondary Electrons
- •References
- •4: X-Rays
- •4.1 Overview
- •4.2 Characteristic X-Rays
- •4.2.1 Origin
- •4.2.2 Fluorescence Yield
- •4.2.3 X-Ray Families
- •4.2.4 X-Ray Nomenclature
- •4.2.6 Characteristic X-Ray Intensity
- •Isolated Atoms
- •X-Ray Production in Thin Foils
- •X-Ray Intensity Emitted from Thick, Solid Specimens
- •4.3 X-Ray Continuum (bremsstrahlung)
- •4.3.1 X-Ray Continuum Intensity
- •4.3.3 Range of X-ray Production
- •4.4 X-Ray Absorption
- •4.5 X-Ray Fluorescence
- •References
- •5.1 Electron Beam Parameters
- •5.2 Electron Optical Parameters
- •5.2.1 Beam Energy
- •Landing Energy
- •5.2.2 Beam Diameter
- •5.2.3 Beam Current
- •5.2.4 Beam Current Density
- •5.2.5 Beam Convergence Angle, α
- •5.2.6 Beam Solid Angle
- •5.2.7 Electron Optical Brightness, β
- •Brightness Equation
- •5.2.8 Focus
- •Astigmatism
- •5.3 SEM Imaging Modes
- •5.3.1 High Depth-of-Field Mode
- •5.3.2 High-Current Mode
- •5.3.3 Resolution Mode
- •5.3.4 Low-Voltage Mode
- •5.4 Electron Detectors
- •5.4.1 Important Properties of BSE and SE for Detector Design and Operation
- •Abundance
- •Angular Distribution
- •Kinetic Energy Response
- •5.4.2 Detector Characteristics
- •Angular Measures for Electron Detectors
- •Elevation (Take-Off) Angle, ψ, and Azimuthal Angle, ζ
- •Solid Angle, Ω
- •Energy Response
- •Bandwidth
- •5.4.3 Common Types of Electron Detectors
- •Backscattered Electrons
- •Passive Detectors
- •Scintillation Detectors
- •Semiconductor BSE Detectors
- •5.4.4 Secondary Electron Detectors
- •Everhart–Thornley Detector
- •Through-the-Lens (TTL) Electron Detectors
- •TTL SE Detector
- •TTL BSE Detector
- •Measuring the DQE: BSE Semiconductor Detector
- •References
- •6: Image Formation
- •6.1 Image Construction by Scanning Action
- •6.2 Magnification
- •6.3 Making Dimensional Measurements With the SEM: How Big Is That Feature?
- •Using a Calibrated Structure in ImageJ-Fiji
- •6.4 Image Defects
- •6.4.1 Projection Distortion (Foreshortening)
- •6.4.2 Image Defocusing (Blurring)
- •6.5 Making Measurements on Surfaces With Arbitrary Topography: Stereomicroscopy
- •6.5.1 Qualitative Stereomicroscopy
- •Fixed beam, Specimen Position Altered
- •Fixed Specimen, Beam Incidence Angle Changed
- •6.5.2 Quantitative Stereomicroscopy
- •Measuring a Simple Vertical Displacement
- •References
- •7: SEM Image Interpretation
- •7.1 Information in SEM Images
- •7.2.2 Calculating Atomic Number Contrast
- •Establishing a Robust Light-Optical Analogy
- •Getting It Wrong: Breaking the Light-Optical Analogy of the Everhart–Thornley (Positive Bias) Detector
- •Deconstructing the SEM/E–T Image of Topography
- •SUM Mode (A + B)
- •DIFFERENCE Mode (A−B)
- •References
- •References
- •9: Image Defects
- •9.1 Charging
- •9.1.1 What Is Specimen Charging?
- •9.1.3 Techniques to Control Charging Artifacts (High Vacuum Instruments)
- •Observing Uncoated Specimens
- •Coating an Insulating Specimen for Charge Dissipation
- •Choosing the Coating for Imaging Morphology
- •9.2 Radiation Damage
- •9.3 Contamination
- •References
- •10: High Resolution Imaging
- •10.2 Instrumentation Considerations
- •10.4.1 SE Range Effects Produce Bright Edges (Isolated Edges)
- •10.4.4 Too Much of a Good Thing: The Bright Edge Effect Hinders Locating the True Position of an Edge for Critical Dimension Metrology
- •10.5.1 Beam Energy Strategies
- •Low Beam Energy Strategy
- •High Beam Energy Strategy
- •Making More SE1: Apply a Thin High-δ Metal Coating
- •Making Fewer BSEs, SE2, and SE3 by Eliminating Bulk Scattering From the Substrate
- •10.6 Factors That Hinder Achieving High Resolution
- •10.6.2 Pathological Specimen Behavior
- •Contamination
- •Instabilities
- •References
- •11: Low Beam Energy SEM
- •11.3 Selecting the Beam Energy to Control the Spatial Sampling of Imaging Signals
- •11.3.1 Low Beam Energy for High Lateral Resolution SEM
- •11.3.2 Low Beam Energy for High Depth Resolution SEM
- •11.3.3 Extremely Low Beam Energy Imaging
- •References
- •12.1.1 Stable Electron Source Operation
- •12.1.2 Maintaining Beam Integrity
- •12.1.4 Minimizing Contamination
- •12.3.1 Control of Specimen Charging
- •12.5 VPSEM Image Resolution
- •References
- •13: ImageJ and Fiji
- •13.1 The ImageJ Universe
- •13.2 Fiji
- •13.3 Plugins
- •13.4 Where to Learn More
- •References
- •14: SEM Imaging Checklist
- •14.1.1 Conducting or Semiconducting Specimens
- •14.1.2 Insulating Specimens
- •14.2 Electron Signals Available
- •14.2.1 Beam Electron Range
- •14.2.2 Backscattered Electrons
- •14.2.3 Secondary Electrons
- •14.3 Selecting the Electron Detector
- •14.3.2 Backscattered Electron Detectors
- •14.3.3 “Through-the-Lens” Detectors
- •14.4 Selecting the Beam Energy for SEM Imaging
- •14.4.4 High Resolution SEM Imaging
- •Strategy 1
- •Strategy 2
- •14.5 Selecting the Beam Current
- •14.5.1 High Resolution Imaging
- •14.5.2 Low Contrast Features Require High Beam Current and/or Long Frame Time to Establish Visibility
- •14.6 Image Presentation
- •14.6.1 “Live” Display Adjustments
- •14.6.2 Post-Collection Processing
- •14.7 Image Interpretation
- •14.7.1 Observer’s Point of View
- •14.7.3 Contrast Encoding
- •14.8.1 VPSEM Advantages
- •14.8.2 VPSEM Disadvantages
- •15: SEM Case Studies
- •15.1 Case Study: How High Is That Feature Relative to Another?
- •15.2 Revealing Shallow Surface Relief
- •16.1.2 Minor Artifacts: The Si-Escape Peak
- •16.1.3 Minor Artifacts: Coincidence Peaks
- •16.1.4 Minor Artifacts: Si Absorption Edge and Si Internal Fluorescence Peak
- •16.2 “Best Practices” for Electron-Excited EDS Operation
- •16.2.1 Operation of the EDS System
- •Choosing the EDS Time Constant (Resolution and Throughput)
- •Choosing the Solid Angle of the EDS
- •Selecting a Beam Current for an Acceptable Level of System Dead-Time
- •16.3.1 Detector Geometry
- •16.3.2 Process Time
- •16.3.3 Optimal Working Distance
- •16.3.4 Detector Orientation
- •16.3.5 Count Rate Linearity
- •16.3.6 Energy Calibration Linearity
- •16.3.7 Other Items
- •16.3.8 Setting Up a Quality Control Program
- •Using the QC Tools Within DTSA-II
- •Creating a QC Project
- •Linearity of Output Count Rate with Live-Time Dose
- •Resolution and Peak Position Stability with Count Rate
- •Solid Angle for Low X-ray Flux
- •Maximizing Throughput at Moderate Resolution
- •References
- •17: DTSA-II EDS Software
- •17.1 Getting Started With NIST DTSA-II
- •17.1.1 Motivation
- •17.1.2 Platform
- •17.1.3 Overview
- •17.1.4 Design
- •Simulation
- •Quantification
- •Experiment Design
- •Modeled Detectors (. Fig. 17.1)
- •Window Type (. Fig. 17.2)
- •The Optimal Working Distance (. Figs. 17.3 and 17.4)
- •Elevation Angle
- •Sample-to-Detector Distance
- •Detector Area
- •Crystal Thickness
- •Number of Channels, Energy Scale, and Zero Offset
- •Resolution at Mn Kα (Approximate)
- •Azimuthal Angle
- •Gold Layer, Aluminum Layer, Nickel Layer
- •Dead Layer
- •Zero Strobe Discriminator (. Figs. 17.7 and 17.8)
- •Material Editor Dialog (. Figs. 17.9, 17.10, 17.11, 17.12, 17.13, and 17.14)
- •17.2.1 Introduction
- •17.2.2 Monte Carlo Simulation
- •17.2.4 Optional Tables
- •References
- •18: Qualitative Elemental Analysis by Energy Dispersive X-Ray Spectrometry
- •18.1 Quality Assurance Issues for Qualitative Analysis: EDS Calibration
- •18.2 Principles of Qualitative EDS Analysis
- •Exciting Characteristic X-Rays
- •Fluorescence Yield
- •X-ray Absorption
- •Si Escape Peak
- •Coincidence Peaks
- •18.3 Performing Manual Qualitative Analysis
- •Beam Energy
- •Choosing the EDS Resolution (Detector Time Constant)
- •Obtaining Adequate Counts
- •18.4.1 Employ the Available Software Tools
- •18.4.3 Lower Photon Energy Region
- •18.4.5 Checking Your Work
- •18.5 A Worked Example of Manual Peak Identification
- •References
- •19.1 What Is a k-ratio?
- •19.3 Sets of k-ratios
- •19.5 The Analytical Total
- •19.6 Normalization
- •19.7.1 Oxygen by Assumed Stoichiometry
- •19.7.3 Element by Difference
- •19.8 Ways of Reporting Composition
- •19.8.1 Mass Fraction
- •19.8.2 Atomic Fraction
- •19.8.3 Stoichiometry
- •19.8.4 Oxide Fractions
- •Example Calculations
- •19.9 The Accuracy of Quantitative Electron-Excited X-ray Microanalysis
- •19.9.1 Standards-Based k-ratio Protocol
- •19.9.2 “Standardless Analysis”
- •19.10 Appendix
- •19.10.1 The Need for Matrix Corrections To Achieve Quantitative Analysis
- •19.10.2 The Physical Origin of Matrix Effects
- •19.10.3 ZAF Factors in Microanalysis
- •X-ray Generation With Depth, φ(ρz)
- •X-ray Absorption Effect, A
- •X-ray Fluorescence, F
- •References
- •20.2 Instrumentation Requirements
- •20.2.1 Choosing the EDS Parameters
- •EDS Spectrum Channel Energy Width and Spectrum Energy Span
- •EDS Time Constant (Resolution and Throughput)
- •EDS Calibration
- •EDS Solid Angle
- •20.2.2 Choosing the Beam Energy, E0
- •20.2.3 Measuring the Beam Current
- •20.2.4 Choosing the Beam Current
- •Optimizing Analysis Strategy
- •20.3.4 Ba-Ti Interference in BaTiSi3O9
- •20.4 The Need for an Iterative Qualitative and Quantitative Analysis Strategy
- •20.4.2 Analysis of a Stainless Steel
- •20.5 Is the Specimen Homogeneous?
- •20.6 Beam-Sensitive Specimens
- •20.6.1 Alkali Element Migration
- •20.6.2 Materials Subject to Mass Loss During Electron Bombardment—the Marshall-Hall Method
- •Thin Section Analysis
- •Bulk Biological and Organic Specimens
- •References
- •21: Trace Analysis by SEM/EDS
- •21.1 Limits of Detection for SEM/EDS Microanalysis
- •21.2.1 Estimating CDL from a Trace or Minor Constituent from Measuring a Known Standard
- •21.2.2 Estimating CDL After Determination of a Minor or Trace Constituent with Severe Peak Interference from a Major Constituent
- •21.3 Measurements of Trace Constituents by Electron-Excited Energy Dispersive X-ray Spectrometry
- •The Inevitable Physics of Remote Excitation Within the Specimen: Secondary Fluorescence Beyond the Electron Interaction Volume
- •Simulation of Long-Range Secondary X-ray Fluorescence
- •NIST DTSA II Simulation: Vertical Interface Between Two Regions of Different Composition in a Flat Bulk Target
- •NIST DTSA II Simulation: Cubic Particle Embedded in a Bulk Matrix
- •21.5 Summary
- •References
- •22.1.2 Low Beam Energy Analysis Range
- •22.2 Advantage of Low Beam Energy X-Ray Microanalysis
- •22.2.1 Improved Spatial Resolution
- •22.3 Challenges and Limitations of Low Beam Energy X-Ray Microanalysis
- •22.3.1 Reduced Access to Elements
- •22.3.3 At Low Beam Energy, Almost Everything Is Found To Be Layered
- •Analysis of Surface Contamination
- •References
- •23: Analysis of Specimens with Special Geometry: Irregular Bulk Objects and Particles
- •23.2.1 No Chemical Etching
- •23.3 Consequences of Attempting Analysis of Bulk Materials With Rough Surfaces
- •23.4.1 The Raw Analytical Total
- •23.4.2 The Shape of the EDS Spectrum
- •23.5 Best Practices for Analysis of Rough Bulk Samples
- •23.6 Particle Analysis
- •Particle Sample Preparation: Bulk Substrate
- •The Importance of Beam Placement
- •Overscanning
- •“Particle Mass Effect”
- •“Particle Absorption Effect”
- •The Analytical Total Reveals the Impact of Particle Effects
- •Does Overscanning Help?
- •23.6.6 Peak-to-Background (P/B) Method
- •Specimen Geometry Severely Affects the k-ratio, but Not the P/B
- •Using the P/B Correspondence
- •23.7 Summary
- •References
- •24: Compositional Mapping
- •24.2 X-Ray Spectrum Imaging
- •24.2.1 Utilizing XSI Datacubes
- •24.2.2 Derived Spectra
- •SUM Spectrum
- •MAXIMUM PIXEL Spectrum
- •24.3 Quantitative Compositional Mapping
- •24.4 Strategy for XSI Elemental Mapping Data Collection
- •24.4.1 Choosing the EDS Dead-Time
- •24.4.2 Choosing the Pixel Density
- •24.4.3 Choosing the Pixel Dwell Time
- •“Flash Mapping”
- •High Count Mapping
- •References
- •25.1 Gas Scattering Effects in the VPSEM
- •25.1.1 Why Doesn’t the EDS Collimator Exclude the Remote Skirt X-Rays?
- •25.2 What Can Be Done To Minimize gas Scattering in VPSEM?
- •25.2.2 Favorable Sample Characteristics
- •Particle Analysis
- •25.2.3 Unfavorable Sample Characteristics
- •References
- •26.1 Instrumentation
- •26.1.2 EDS Detector
- •26.1.3 Probe Current Measurement Device
- •Direct Measurement: Using a Faraday Cup and Picoammeter
- •A Faraday Cup
- •Electrically Isolated Stage
- •Indirect Measurement: Using a Calibration Spectrum
- •26.1.4 Conductive Coating
- •26.2 Sample Preparation
- •26.2.1 Standard Materials
- •26.2.2 Peak Reference Materials
- •26.3 Initial Set-Up
- •26.3.1 Calibrating the EDS Detector
- •Selecting a Pulse Process Time Constant
- •Energy Calibration
- •Quality Control
- •Sample Orientation
- •Detector Position
- •Probe Current
- •26.4 Collecting Data
- •26.4.1 Exploratory Spectrum
- •26.4.2 Experiment Optimization
- •26.4.3 Selecting Standards
- •26.4.4 Reference Spectra
- •26.4.5 Collecting Standards
- •26.4.6 Collecting Peak-Fitting References
- •26.5 Data Analysis
- •26.5.2 Quantification
- •26.6 Quality Check
- •Reference
- •27.2 Case Study: Aluminum Wire Failures in Residential Wiring
- •References
- •28: Cathodoluminescence
- •28.1 Origin
- •28.2 Measuring Cathodoluminescence
- •28.3 Applications of CL
- •28.3.1 Geology
- •Carbonado Diamond
- •Ancient Impact Zircons
- •28.3.2 Materials Science
- •Semiconductors
- •Lead-Acid Battery Plate Reactions
- •28.3.3 Organic Compounds
- •References
- •29.1.1 Single Crystals
- •29.1.2 Polycrystalline Materials
- •29.1.3 Conditions for Detecting Electron Channeling Contrast
- •Specimen Preparation
- •Instrument Conditions
- •29.2.1 Origin of EBSD Patterns
- •29.2.2 Cameras for EBSD Pattern Detection
- •29.2.3 EBSD Spatial Resolution
- •29.2.5 Steps in Typical EBSD Measurements
- •Sample Preparation for EBSD
- •Align Sample in the SEM
- •Check for EBSD Patterns
- •Adjust SEM and Select EBSD Map Parameters
- •Run the Automated Map
- •29.2.6 Display of the Acquired Data
- •29.2.7 Other Map Components
- •29.2.10 Application Example
- •Application of EBSD To Understand Meteorite Formation
- •29.2.11 Summary
- •Specimen Considerations
- •EBSD Detector
- •Selection of Candidate Crystallographic Phases
- •Microscope Operating Conditions and Pattern Optimization
- •Selection of EBSD Acquisition Parameters
- •Collect the Orientation Map
- •References
- •30.1 Introduction
- •30.2 Ion–Solid Interactions
- •30.3 Focused Ion Beam Systems
- •30.5 Preparation of Samples for SEM
- •30.5.1 Cross-Section Preparation
- •30.5.2 FIB Sample Preparation for 3D Techniques and Imaging
- •30.6 Summary
- •References
- •31: Ion Beam Microscopy
- •31.1 What Is So Useful About Ions?
- •31.2 Generating Ion Beams
- •31.3 Signal Generation in the HIM
- •31.5 Patterning with Ion Beams
- •31.7 Chemical Microanalysis with Ion Beams
- •References
- •Appendix
- •A Database of Electron–Solid Interactions
- •A Database of Electron–Solid Interactions
- •Introduction
- •Backscattered Electrons
- •Secondary Yields
- •Stopping Powers
- •X-ray Ionization Cross Sections
- •Conclusions
- •References
- •Index
- •Reference List
- •Index
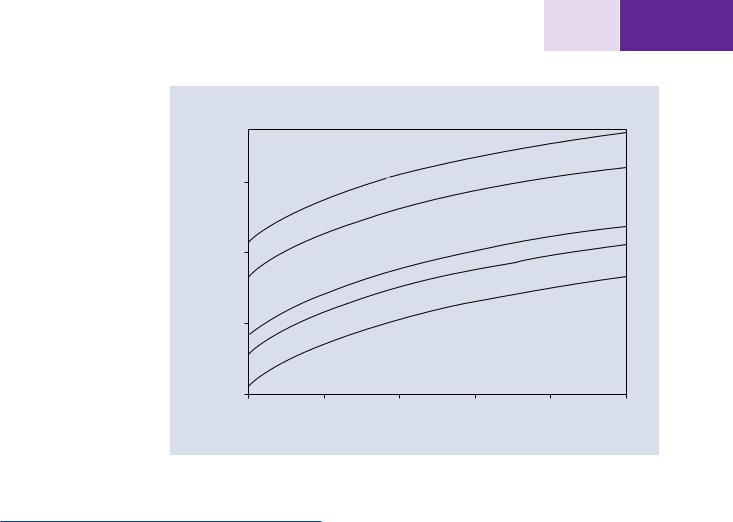
5 |
1 |
1.4 · Simulating the Effects of Elastic Scattering: Monte Carlo Calculations
. Fig. 1.4 Elastic mean free path as a function of electron kinetic energy for various elements
Elastic mean free path (nm)
10
1
0.1
0.01
5
Elastic scattering mean free path (f0 = 2°)
C
Al
Cu
Ag
Au
10 |
15 |
20 |
25 |
30 |
|
Beam energy (keV) |
|
|
1.4\ Simulating the Effects of Elastic
Scattering: Monte Carlo Calculations
Inelastic scattering sets a limit on the total distance traveled by the beam electron. The Bethe range is an estimate of this distance and can be found by integrating the Bethe continuous energy loss expression from the incident beam energy E0 down to a low energy limit, for example, 2 keV. Estimating the effects of elastic scattering on the beam electrons is much more complicated. Any individual elastic scattering event can result in a scattering angle within a broad range from a threshold of a fraction of a degree up to 180°, with small scattering angles much more likely than very large values and an average value typically in the range 5–10°. Moreover, the electron scattered by the atom through an angle ϕ in
. Fig. 1.3a at point P1 can actually follow any path along the surface of the three-dimensional scattering cone shown in
. Fig. 1.3b and can land anywhere in the circumference of the base of the scattering cone (i.e., the azimuthal angle in the base of the cone ranges from 0 to 360° with equal probability), resulting in a three-dimensional path. The length of the trajectory along the surface of the scattering cone depends on the frequency of elastic events with distance traveled and can be estimated from Eq. 1.3a for the elastic
scattering mean free path, λelastic. The next elastic scattering event P2 causes the electron to deviate in a new direction, as
shown in . Fig. 1.3c, creating an increasingly complex path. Because of the random component of scattering at each of many steps, this complex behavior cannot be adequately described by an algebraic expression like the Bethe continuous energy loss equation. Instead, a stepwise simulation of the electron's behavior must be constructed that incorporates inelastic and elastic scattering. Several simplifications are introduced to create a practical “Monte Carlo electron trajectory simulation”:
\1.\ All of the angular deviation of the beam electron is ascribed to elastic scattering. A mathematical model for elastic scattering is applied that utilizes a random number (hence the name “Monte Carlo” from the supposed randomness of gambling) to select a properly weighted value of the elastic scattering angle out of the possible range (from a threshold value of approximately 1° to a maximum of 180°). A second random number is used to select the azimuthal angle in the base of the scattering cone in . Fig. 1.1c.
\2.\ The distance between elastic scattering events, s, which lies on the surface of the scattering cone in . Fig. 1.3b, is calculated from the elastic mean free path, Eq. 1.3b.
\3.\ Inelastic scattering is calculated with the Bethe continuous energy loss expression, Eq. 1.1b. The specific energy loss, E, along the path, s, in the surface of the scattering cone, . Fig. 1.3b, is calculated with the Bethe continuous energy loss expression: E = (dE/ds)*s
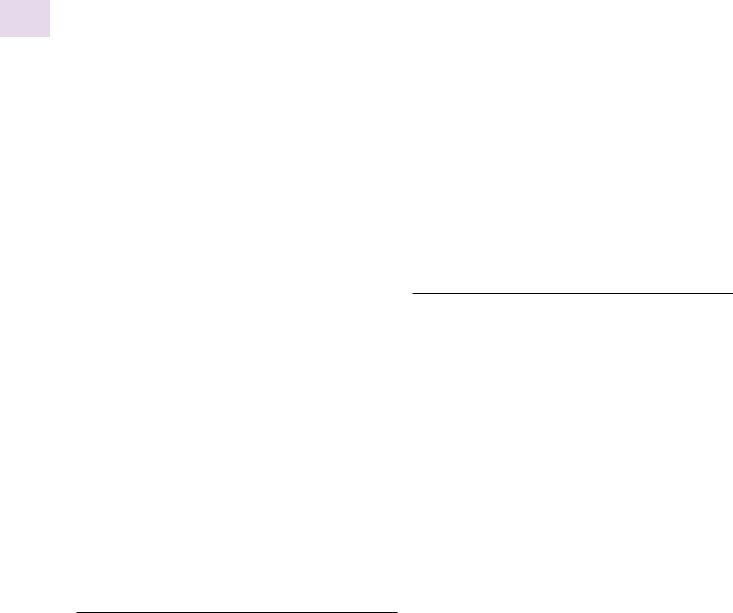
\6 Chapter 1 · Electron Beam—Specimen Interactions: Interaction Volume
Given a specific set of these parameters, the Monte Carlo elec- 1 tron trajectory simulation utilizes geometrical expressions to calculate the successive series of locations P1, P2, P3, etc., successively determining the coordinate locations (x, y, z) that the energetic electron follows within the solid. At each location P, the newly depreciated energy of the electron is known, and after the next elastic scattering angle is calculated, the new velocity vector components vx, vy, vz are determined to transport the electron to the next location. A trajectory ends when either the electron energy falls below a threshold of interest (e.g., 1 keV), or else the path takes it outside the geometric bounds of the specimen, which is determined by comparing the current location (x, y, z) with the specimen boundaries. The capability of simulating electron beam interactions in specimens with complex geometrical shapes is one of the major strengths of the
Monte Carlo electron trajectory simulation method.
Monte Carlo electron trajectory simulation can provide visual depictions as well as numerical results of the beam–specimen interaction, creating a powerful instructional tool for studying this complex phenomenon. Several powerful Monte Carlo simulations appropriate for SEM and X-ray microanalysis applications are available as free resources:
CASINO [7http://www.gel.usherbrooke.ca/casino/What.html] Joy Monte Carlo [7http://web.utk.edu/~srcutk/htm/
simulati.htm]
NIST DTSA-II [7http://www.cstl.nist.gov/div837/837.02/ epq/dtsa2/index.html]
While the static images of Monte Carlo simulations presented below are useful instructional aids, readers are encouraged to perform their own simulations to become familiar with this powerful tool, which in more elaborate implementations is an important aid in understanding critical aspects of SEM imaging.
1.4.1\ What Do Individual Monte Carlo
Trajectories Look Like?
Perform a Monte Carlo simulation (CASINO simulation) for copper with a beam energy of 20 keV and a tilt of 0° (beam perpendicular to the surface) for a small number of trajectories, for example, 25. . Figure 1.5a, b show two simulations of 25 trajectories each. The trajectories are actually determined in three dimensions (x-y-z, where x-y defines the surface plane and z is perpendicular to the surface) but for plotting are rendered in two dimensions (x-z), with the third
dimension y projected onto the x-z plane. (An example of the true three-dimensional trajectories, simulated with the Joy Monte Carlo, is shown in . Fig. 1.6, in which a small number of trajectories (to minimize overlap) have been rendered as an anaglyph stereo representation with the convention left eye = red filter. Inspection of this simulation shows the y motion of the electrons in and out of the x-z plane.) The stochastic nature of the interaction imposed by the nature of elastic scattering is readily apparent in the great variation among the individual trajectories seen in . Fig. 1.5a, b. It quickly becomes clear that individual beam electrons follow a huge range of paths and simulating a small number of trajectories does not provide an adequate view of the electron beam specimen interaction.
1.4.2\ Monte Carlo Simulation To Visualize
the Electron Interaction Volume
To capture a reasonable picture representation of the electron interaction volume, which is the region of the specimen in which the beam electrons travel and deposit energy, it is necessary to calculate many more trajectories. . Figure 1.5c shows the simulation for copper, E0 = 20 keV at 0° tilt extended to 500 trajectories, which reveals the full extent of the electron interaction volume. Beyond a few hundred trajectories, superimposing the three-dimensional trajectories to create a two-dimensional representation reaches diminishing returns due to overlap of the plotted lines. While simulating 500 trajectories provides a reasonable qualitative view of the electron interaction volume, Monte Carlo calculations of numerical properties of the interaction volume and related processes, such as electron backscattering (discussed in the backscattered electron module), are subject to statistically predictable variations because of the use of random numbers to select the elastic scattering parameters. Variance in repeated simulations of the same starting conditions is related to the number of trajectories and can be described with the properties of the Gaussian (normal) distribution. Thus the precision, p, of the calculation of a parameter of the interaction is related to the total number of simulated trajectories, n, and the fraction, f, of those trajectories that produce the effect of interest (e.g., backscattering):
p = ( f n)1/ 2 / ( f n)= ( f n)−1/ 2 |
\ |
(1.4) |
|
|
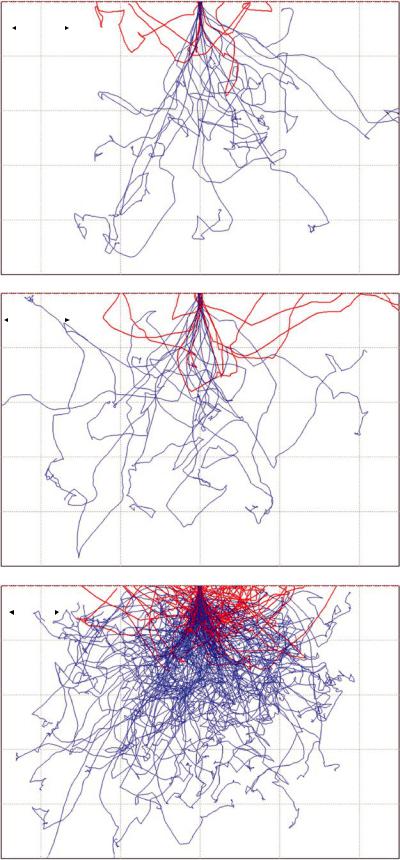
1.4 · Simulating the Effects of Elastic Scattering: Monte Carlo Calculations |
|
|
|
|
7 |
|
|
1 |
||||||||||
|
|
|
|
|
|
|
|
|||||||||||
|
|
|
|
|
|
|
|
|||||||||||
. Fig. 1.5 a Copper, E0 = 20 keV; 0 tilt; 25 trajec- |
a |
|
|
|
|
|
|
|
|
|||||||||
tories (CASINO Monte Carlo simultion). b Copper, |
Cu E0 = 20 keV |
|
|
|
|
|
|
0.0 nm |
||||||||||
E0 = 20 keV; 0 tilt; another 25 trajectories. c Copper, |
|
|
|
|
|
|
|
|
||||||||||
|
|
|
|
|
|
|
|
|
|
|
|
|
|
|
|
|
||
E0 = 20 keV; 0 tilt; 200 trajectories |
|
|
|
|
|
|
|
|
|
|
|
|
|
|
|
|
|
|
|
|
|
|
200 nm |
|
|
|
|
|
|
|
|
||||||
|
|
|
|
|
|
|
|
|
|
|
|
|
|
|
|
200.0 nm |
||
|
|
|
|
|
|
|
|
|
|
|
|
|
|
|
|
400.0 nm |
||
|
|
|
|
|
|
|
|
|
|
|
|
|
|
|
|
600.0 nm |
||
|
|
|
|
|
|
|
|
|
|
|
|
|
|
|
|
800.0 nm |
||
|
|
|
-582.5 nm |
|
-291.3 nm |
-0.0 nm |
291.3 nm |
582.5 nm |
||||||||||
|
|
b |
|
|
|
|
|
|
0.0 nm |
|||||||||
|
|
Cu E0 = 20 keV |
|
|
|
|
|
|
||||||||||
|
|
|
|
|
|
|
|
|
|
|||||||||
|
|
|
|
|
|
|
|
|
|
|
|
|
|
|
|
|||
|
|
|
|
|
|
200 nm |
|
|
|
|
|
|
|
|
|
|
||
|
|
|
|
|
|
|
|
|
|
|
|
|
|
|
|
180.0 nm |
||
|
|
|
|
|
|
|
|
|
|
|
|
|
|
|
|
|||
|
|
|
|
|
|
|
|
|
|
|
|
|
|
|
|
360.0 nm |
||
|
|
|
|
|
|
|
|
|
|
|
|
|
|
|
|
540.0 nm |
||
|
|
|
|
|
|
|
|
|
|
|
|
|
|
|
|
720.0 nm |
||
|
|
|
|
|
-524.3 nm |
|
-262.1 nm |
-0.0 nm |
262.1 nm |
524.3 nm |
||||||||
|
|
c |
|
|
|
|
|
|
0.0 nm |
|||||||||
|
|
|
|
|
|
|
|
|
|
|
|
|
|
|
|
|
||
|
|
Cu E0 = 20 keV |
|
|
|
|
|
|
||||||||||
|
|
|
|
|
|
|
|
|
|
|||||||||
|
|
|
|
|
|
|
|
|
|
|
|
|
|
|
|
|
|
|
|
|
|
|
|
200 nm |
|
|
|
|
|
|
|
|
|
|
|
||
|
|
|
|
|
|
|
|
|
|
|
|
|
|
|
|
233.5 nm |
||
|
|
|
|
|
|
|
|
|
|
|
|
|
|
|
|
|||
|
|
|
|
|
|
|
|
|
|
|
|
|
|
|
|
466.9 nm |
||
|
|
|
|
|
|
|
|
|
|
|
|
|
|
|
|
700.4 nm |
||
|
|
|
|
|
|
|
|
|
|
|
|
|
|
|
|
933.8 nm |
||
|
|
|
|
|
-680.0 nm |
|
-340.0 nm |
-0.0 nm |
340.0 nm |
680.0 nm |

\8 Chapter 1 · Electron Beam—Specimen Interactions: Interaction Volume
1
Energy (keV) 20
Tilt/TOA 0
Number 35
Select
500 nm
Repeat
computed BS yield = 0.31 |
Exit |
. Fig. 1.6 Three-dimensional representation of a Monte Carlo simulation (Cu, 20 keV, 0° tilt) using the anaglyph stereo method (left eye = red filter) (Joy Monte Carlo)
1.4.3\ Using the Monte Carlo Electron
Trajectory Simulation to Study
the Interaction Volume
What Are the Main Features of the Beam Electron Interaction Volume?
In . Fig. 1.5c, the beam electron interaction volume is seen to be a very complex structure with dimensions extending over hundreds to thousands of nanometers from the beam impact point, depending on target material and the beam energy. At 0° tilt, the interaction volume is rotationally symmetric around the beam. While the electron trajectories provide a strong visual representation of the interaction volume, more informative numerical information is needed. The Monte Carlo simulation can provide detailed information on many aspects of the electron beam–specimen interaction. The color-encoding of the energy deposited along each trajectory, as implemented in the Joy Monte Carlo shown in . Fig. 1.11, creates a view that reveals the general three-dimensional complexity of energy deposition within the interaction volume. The CASINO Monte Carlo provides an even more detailed view of energy deposition, as shown in . Fig. 1.7. The energy deposition per unit volume is greatest just under the beam impact location and rapidly falls off as the periphery of the interaction volume is approached. This calculation reveals that a small cylindrical volume under the beam impact point, shown in more detail in . Fig. 1.7b, receives half of the total energy deposited by the beam in the specimen (that is, the volume within the 50% contour), with the
balance of the energy deposited in a strongly non-linear fashion in the much larger portion of the interaction volume.
How Does the Interaction Volume Change with Composition?
. Figure 1.8 shows the interaction volume in various targets, C, Si, Cu, Ag, and Au, at fixed beam energy, E0 = 20 keV, and 0° tilt. As the atomic number of the target increases, the linear dimensions of the interaction volume decrease. The form also changes from pear-shaped with a dense conical region below the beam impact for low atomic number targets to a more hemispherical shape for high atomic number targets.
kNote the dramatic change of scale
Approximately 12 gold atoms were encountered within the footprint of a 1-nm diameter at the surface. Without considering the effects of elastic scattering, the Bethe range for Au at an incident beam energy of 20 keV limited the penetration of the beam to approximately 1200 nm and a cylindrical volume of approximately 940 nm3, containing approximately 5.6 × 104 Au atoms. The effect of elastic scattering is to create a three-dimensional hemispherical interaction volume with a radius of approximately 600 nm and a volume of 4.5 × 108 nm3, containing 2.7 × 1010 Au atoms, an increase of nine orders-of-magnitude over the number of atoms encountered in the initial beam footprint on the surface.
How Does the Interaction Volume Change with Incident Beam Energy?
. Figure 1.9 shows the interaction volume for copper at 0° tilt over a range of incident beam energy from 5 to 30 keV. The shape of the interaction volume is relatively independent of beam energy, but the size increases rapidly as the incident beam energy increases.
How Does the Interaction Volume Change with Specimen Tilt?
. Figure 1.10 shows the interaction volume for copper at an incident beam energy of 20 keV and a series of tilt angles. As the tilt angle increases so that the beam approaches the surface at a progressively more shallow angle, the shape of the interaction volume changes significantly. At 0° tilt, the interaction volume is rotationally symmetric around the beam, but as the tilt angle increases the interaction volume becomes asymmetric, with the dense portion of the distribution shifting progressively away from the beam impact point. The maximum penetration of the beam is reduced as the tilt angle increases.

1.4 · Simulating the Effects of Elastic Scattering: Monte Carlo Calculations
. Fig. 1.7 a Isocontours of |
a |
|
|
energy loss showing fraction |
|
|
|
Cu E0 |
= 20 keV |
|
|
remaining; Cu, 20 keV, 0° tilt; |
90% |
||
50,000 trajectories (CASINO Monte |
|
|
|
|
|
|
Carlo simulation). b Expanded |
75% |
|
view of high density region of 1.7a |
||
50% |
||
|
||
|
25% |
|
|
10% |
|
|
5% |
|
|
5.0% |
|
|
10.0% |
|
|
25.0% |
|
|
50.0% |
|
|
75.0% |
|
|
90.0% |
91
0.0nm
206.3nm
412.6nm
618.9nm
825.2nm
-600.9 nm |
-300.4 nm |
0.0 nm |
300.4 nm |
600.9 nm |
b
10%
90%
25% 75%
50%
200 nm

\10 |
|
Chapter 1 · Electron Beam—Specimen Interactions: Interaction Volume |
|||
|
|
|
|
0.0 nm |
|
1 |
|
|
|
||
|
|
C |
|||
|
|
|
Cu |
||
|
|
|
|
|
|
|
|
|
|
|
|
|
|
|
|
|
|
|
|
|
|
892.6 nm |
1785.3 nm
Ag
2677.9 nm
|
|
|
|
3570.6 nm |
|
-2600.0 nm |
-1300.0 nm |
-0.0 nm |
1300.0 nm |
2600.0 nm |
Au |
0.0 nm
Si
755.3 nm
E0= 20 keV 0° tilt
1510.6 nm
1 µm
2265.9 nm
3021.3 nm
-2200.0 nm |
-1100.0 nm |
-0.0 nm |
1100.0 nm |
2200.0 nm |
. Fig. 1.8 Monte Carlo simulations for an incident beam energy of 20 keV and 0° tilt for C, Si, Cu, Ag, and Au, all shown at the same scale (CASINO Monte Carlo simulation)
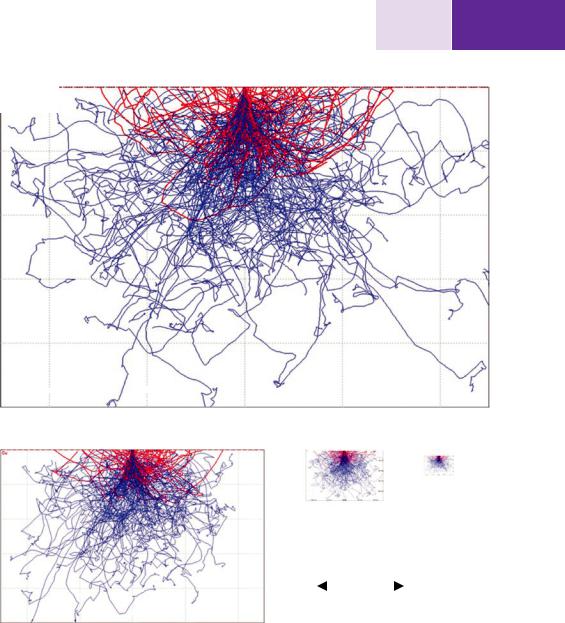
1.4 · Simulating the Effects of Elastic Scattering: Monte Carlo Calculations
. Fig. 1.9 Monte Carlo simula-
tions for Cu, 0° tilt, incident beam 30 keV energies 5, 10, 20, and 30 keV
(CASINO Monte Carlo simulation)
-1260.0 nm -630.0 nm 0.0 nm 630.0 nm
10 keV
|
|
|
|
0.0 nm |
|
20 keV |
|
|
|
|
|
|
|
|
|
233.5 nm |
|
|
|
|
|
|
|
|
|
|
|
466.9 nm |
|
|
|
|
|
700.4 nm |
Cu |
|
|
|
|
|
|
|
|
|
|
|
0° tilt |
|
|
|
|
933.8 nm |
|
|
|
|
|
500 nm |
|
|
|
|
|
|
|
-680.0 nm |
-340.0 nm |
0.0 nm |
340.0 nm |
680.0 nm |
|
111
0.0nm
432.6 nm
865.2 nm
1297.8 nm
1730.4 nm
1260.0 nm
5 keV