
- •Preface
- •Imaging Microscopic Features
- •Measuring the Crystal Structure
- •References
- •Contents
- •1.4 Simulating the Effects of Elastic Scattering: Monte Carlo Calculations
- •What Are the Main Features of the Beam Electron Interaction Volume?
- •How Does the Interaction Volume Change with Composition?
- •How Does the Interaction Volume Change with Incident Beam Energy?
- •How Does the Interaction Volume Change with Specimen Tilt?
- •1.5 A Range Equation To Estimate the Size of the Interaction Volume
- •References
- •2: Backscattered Electrons
- •2.1 Origin
- •2.2.1 BSE Response to Specimen Composition (η vs. Atomic Number, Z)
- •SEM Image Contrast with BSE: “Atomic Number Contrast”
- •SEM Image Contrast: “BSE Topographic Contrast—Number Effects”
- •2.2.3 Angular Distribution of Backscattering
- •Beam Incident at an Acute Angle to the Specimen Surface (Specimen Tilt > 0°)
- •SEM Image Contrast: “BSE Topographic Contrast—Trajectory Effects”
- •2.2.4 Spatial Distribution of Backscattering
- •Depth Distribution of Backscattering
- •Radial Distribution of Backscattered Electrons
- •2.3 Summary
- •References
- •3: Secondary Electrons
- •3.1 Origin
- •3.2 Energy Distribution
- •3.3 Escape Depth of Secondary Electrons
- •3.8 Spatial Characteristics of Secondary Electrons
- •References
- •4: X-Rays
- •4.1 Overview
- •4.2 Characteristic X-Rays
- •4.2.1 Origin
- •4.2.2 Fluorescence Yield
- •4.2.3 X-Ray Families
- •4.2.4 X-Ray Nomenclature
- •4.2.6 Characteristic X-Ray Intensity
- •Isolated Atoms
- •X-Ray Production in Thin Foils
- •X-Ray Intensity Emitted from Thick, Solid Specimens
- •4.3 X-Ray Continuum (bremsstrahlung)
- •4.3.1 X-Ray Continuum Intensity
- •4.3.3 Range of X-ray Production
- •4.4 X-Ray Absorption
- •4.5 X-Ray Fluorescence
- •References
- •5.1 Electron Beam Parameters
- •5.2 Electron Optical Parameters
- •5.2.1 Beam Energy
- •Landing Energy
- •5.2.2 Beam Diameter
- •5.2.3 Beam Current
- •5.2.4 Beam Current Density
- •5.2.5 Beam Convergence Angle, α
- •5.2.6 Beam Solid Angle
- •5.2.7 Electron Optical Brightness, β
- •Brightness Equation
- •5.2.8 Focus
- •Astigmatism
- •5.3 SEM Imaging Modes
- •5.3.1 High Depth-of-Field Mode
- •5.3.2 High-Current Mode
- •5.3.3 Resolution Mode
- •5.3.4 Low-Voltage Mode
- •5.4 Electron Detectors
- •5.4.1 Important Properties of BSE and SE for Detector Design and Operation
- •Abundance
- •Angular Distribution
- •Kinetic Energy Response
- •5.4.2 Detector Characteristics
- •Angular Measures for Electron Detectors
- •Elevation (Take-Off) Angle, ψ, and Azimuthal Angle, ζ
- •Solid Angle, Ω
- •Energy Response
- •Bandwidth
- •5.4.3 Common Types of Electron Detectors
- •Backscattered Electrons
- •Passive Detectors
- •Scintillation Detectors
- •Semiconductor BSE Detectors
- •5.4.4 Secondary Electron Detectors
- •Everhart–Thornley Detector
- •Through-the-Lens (TTL) Electron Detectors
- •TTL SE Detector
- •TTL BSE Detector
- •Measuring the DQE: BSE Semiconductor Detector
- •References
- •6: Image Formation
- •6.1 Image Construction by Scanning Action
- •6.2 Magnification
- •6.3 Making Dimensional Measurements With the SEM: How Big Is That Feature?
- •Using a Calibrated Structure in ImageJ-Fiji
- •6.4 Image Defects
- •6.4.1 Projection Distortion (Foreshortening)
- •6.4.2 Image Defocusing (Blurring)
- •6.5 Making Measurements on Surfaces With Arbitrary Topography: Stereomicroscopy
- •6.5.1 Qualitative Stereomicroscopy
- •Fixed beam, Specimen Position Altered
- •Fixed Specimen, Beam Incidence Angle Changed
- •6.5.2 Quantitative Stereomicroscopy
- •Measuring a Simple Vertical Displacement
- •References
- •7: SEM Image Interpretation
- •7.1 Information in SEM Images
- •7.2.2 Calculating Atomic Number Contrast
- •Establishing a Robust Light-Optical Analogy
- •Getting It Wrong: Breaking the Light-Optical Analogy of the Everhart–Thornley (Positive Bias) Detector
- •Deconstructing the SEM/E–T Image of Topography
- •SUM Mode (A + B)
- •DIFFERENCE Mode (A−B)
- •References
- •References
- •9: Image Defects
- •9.1 Charging
- •9.1.1 What Is Specimen Charging?
- •9.1.3 Techniques to Control Charging Artifacts (High Vacuum Instruments)
- •Observing Uncoated Specimens
- •Coating an Insulating Specimen for Charge Dissipation
- •Choosing the Coating for Imaging Morphology
- •9.2 Radiation Damage
- •9.3 Contamination
- •References
- •10: High Resolution Imaging
- •10.2 Instrumentation Considerations
- •10.4.1 SE Range Effects Produce Bright Edges (Isolated Edges)
- •10.4.4 Too Much of a Good Thing: The Bright Edge Effect Hinders Locating the True Position of an Edge for Critical Dimension Metrology
- •10.5.1 Beam Energy Strategies
- •Low Beam Energy Strategy
- •High Beam Energy Strategy
- •Making More SE1: Apply a Thin High-δ Metal Coating
- •Making Fewer BSEs, SE2, and SE3 by Eliminating Bulk Scattering From the Substrate
- •10.6 Factors That Hinder Achieving High Resolution
- •10.6.2 Pathological Specimen Behavior
- •Contamination
- •Instabilities
- •References
- •11: Low Beam Energy SEM
- •11.3 Selecting the Beam Energy to Control the Spatial Sampling of Imaging Signals
- •11.3.1 Low Beam Energy for High Lateral Resolution SEM
- •11.3.2 Low Beam Energy for High Depth Resolution SEM
- •11.3.3 Extremely Low Beam Energy Imaging
- •References
- •12.1.1 Stable Electron Source Operation
- •12.1.2 Maintaining Beam Integrity
- •12.1.4 Minimizing Contamination
- •12.3.1 Control of Specimen Charging
- •12.5 VPSEM Image Resolution
- •References
- •13: ImageJ and Fiji
- •13.1 The ImageJ Universe
- •13.2 Fiji
- •13.3 Plugins
- •13.4 Where to Learn More
- •References
- •14: SEM Imaging Checklist
- •14.1.1 Conducting or Semiconducting Specimens
- •14.1.2 Insulating Specimens
- •14.2 Electron Signals Available
- •14.2.1 Beam Electron Range
- •14.2.2 Backscattered Electrons
- •14.2.3 Secondary Electrons
- •14.3 Selecting the Electron Detector
- •14.3.2 Backscattered Electron Detectors
- •14.3.3 “Through-the-Lens” Detectors
- •14.4 Selecting the Beam Energy for SEM Imaging
- •14.4.4 High Resolution SEM Imaging
- •Strategy 1
- •Strategy 2
- •14.5 Selecting the Beam Current
- •14.5.1 High Resolution Imaging
- •14.5.2 Low Contrast Features Require High Beam Current and/or Long Frame Time to Establish Visibility
- •14.6 Image Presentation
- •14.6.1 “Live” Display Adjustments
- •14.6.2 Post-Collection Processing
- •14.7 Image Interpretation
- •14.7.1 Observer’s Point of View
- •14.7.3 Contrast Encoding
- •14.8.1 VPSEM Advantages
- •14.8.2 VPSEM Disadvantages
- •15: SEM Case Studies
- •15.1 Case Study: How High Is That Feature Relative to Another?
- •15.2 Revealing Shallow Surface Relief
- •16.1.2 Minor Artifacts: The Si-Escape Peak
- •16.1.3 Minor Artifacts: Coincidence Peaks
- •16.1.4 Minor Artifacts: Si Absorption Edge and Si Internal Fluorescence Peak
- •16.2 “Best Practices” for Electron-Excited EDS Operation
- •16.2.1 Operation of the EDS System
- •Choosing the EDS Time Constant (Resolution and Throughput)
- •Choosing the Solid Angle of the EDS
- •Selecting a Beam Current for an Acceptable Level of System Dead-Time
- •16.3.1 Detector Geometry
- •16.3.2 Process Time
- •16.3.3 Optimal Working Distance
- •16.3.4 Detector Orientation
- •16.3.5 Count Rate Linearity
- •16.3.6 Energy Calibration Linearity
- •16.3.7 Other Items
- •16.3.8 Setting Up a Quality Control Program
- •Using the QC Tools Within DTSA-II
- •Creating a QC Project
- •Linearity of Output Count Rate with Live-Time Dose
- •Resolution and Peak Position Stability with Count Rate
- •Solid Angle for Low X-ray Flux
- •Maximizing Throughput at Moderate Resolution
- •References
- •17: DTSA-II EDS Software
- •17.1 Getting Started With NIST DTSA-II
- •17.1.1 Motivation
- •17.1.2 Platform
- •17.1.3 Overview
- •17.1.4 Design
- •Simulation
- •Quantification
- •Experiment Design
- •Modeled Detectors (. Fig. 17.1)
- •Window Type (. Fig. 17.2)
- •The Optimal Working Distance (. Figs. 17.3 and 17.4)
- •Elevation Angle
- •Sample-to-Detector Distance
- •Detector Area
- •Crystal Thickness
- •Number of Channels, Energy Scale, and Zero Offset
- •Resolution at Mn Kα (Approximate)
- •Azimuthal Angle
- •Gold Layer, Aluminum Layer, Nickel Layer
- •Dead Layer
- •Zero Strobe Discriminator (. Figs. 17.7 and 17.8)
- •Material Editor Dialog (. Figs. 17.9, 17.10, 17.11, 17.12, 17.13, and 17.14)
- •17.2.1 Introduction
- •17.2.2 Monte Carlo Simulation
- •17.2.4 Optional Tables
- •References
- •18: Qualitative Elemental Analysis by Energy Dispersive X-Ray Spectrometry
- •18.1 Quality Assurance Issues for Qualitative Analysis: EDS Calibration
- •18.2 Principles of Qualitative EDS Analysis
- •Exciting Characteristic X-Rays
- •Fluorescence Yield
- •X-ray Absorption
- •Si Escape Peak
- •Coincidence Peaks
- •18.3 Performing Manual Qualitative Analysis
- •Beam Energy
- •Choosing the EDS Resolution (Detector Time Constant)
- •Obtaining Adequate Counts
- •18.4.1 Employ the Available Software Tools
- •18.4.3 Lower Photon Energy Region
- •18.4.5 Checking Your Work
- •18.5 A Worked Example of Manual Peak Identification
- •References
- •19.1 What Is a k-ratio?
- •19.3 Sets of k-ratios
- •19.5 The Analytical Total
- •19.6 Normalization
- •19.7.1 Oxygen by Assumed Stoichiometry
- •19.7.3 Element by Difference
- •19.8 Ways of Reporting Composition
- •19.8.1 Mass Fraction
- •19.8.2 Atomic Fraction
- •19.8.3 Stoichiometry
- •19.8.4 Oxide Fractions
- •Example Calculations
- •19.9 The Accuracy of Quantitative Electron-Excited X-ray Microanalysis
- •19.9.1 Standards-Based k-ratio Protocol
- •19.9.2 “Standardless Analysis”
- •19.10 Appendix
- •19.10.1 The Need for Matrix Corrections To Achieve Quantitative Analysis
- •19.10.2 The Physical Origin of Matrix Effects
- •19.10.3 ZAF Factors in Microanalysis
- •X-ray Generation With Depth, φ(ρz)
- •X-ray Absorption Effect, A
- •X-ray Fluorescence, F
- •References
- •20.2 Instrumentation Requirements
- •20.2.1 Choosing the EDS Parameters
- •EDS Spectrum Channel Energy Width and Spectrum Energy Span
- •EDS Time Constant (Resolution and Throughput)
- •EDS Calibration
- •EDS Solid Angle
- •20.2.2 Choosing the Beam Energy, E0
- •20.2.3 Measuring the Beam Current
- •20.2.4 Choosing the Beam Current
- •Optimizing Analysis Strategy
- •20.3.4 Ba-Ti Interference in BaTiSi3O9
- •20.4 The Need for an Iterative Qualitative and Quantitative Analysis Strategy
- •20.4.2 Analysis of a Stainless Steel
- •20.5 Is the Specimen Homogeneous?
- •20.6 Beam-Sensitive Specimens
- •20.6.1 Alkali Element Migration
- •20.6.2 Materials Subject to Mass Loss During Electron Bombardment—the Marshall-Hall Method
- •Thin Section Analysis
- •Bulk Biological and Organic Specimens
- •References
- •21: Trace Analysis by SEM/EDS
- •21.1 Limits of Detection for SEM/EDS Microanalysis
- •21.2.1 Estimating CDL from a Trace or Minor Constituent from Measuring a Known Standard
- •21.2.2 Estimating CDL After Determination of a Minor or Trace Constituent with Severe Peak Interference from a Major Constituent
- •21.3 Measurements of Trace Constituents by Electron-Excited Energy Dispersive X-ray Spectrometry
- •The Inevitable Physics of Remote Excitation Within the Specimen: Secondary Fluorescence Beyond the Electron Interaction Volume
- •Simulation of Long-Range Secondary X-ray Fluorescence
- •NIST DTSA II Simulation: Vertical Interface Between Two Regions of Different Composition in a Flat Bulk Target
- •NIST DTSA II Simulation: Cubic Particle Embedded in a Bulk Matrix
- •21.5 Summary
- •References
- •22.1.2 Low Beam Energy Analysis Range
- •22.2 Advantage of Low Beam Energy X-Ray Microanalysis
- •22.2.1 Improved Spatial Resolution
- •22.3 Challenges and Limitations of Low Beam Energy X-Ray Microanalysis
- •22.3.1 Reduced Access to Elements
- •22.3.3 At Low Beam Energy, Almost Everything Is Found To Be Layered
- •Analysis of Surface Contamination
- •References
- •23: Analysis of Specimens with Special Geometry: Irregular Bulk Objects and Particles
- •23.2.1 No Chemical Etching
- •23.3 Consequences of Attempting Analysis of Bulk Materials With Rough Surfaces
- •23.4.1 The Raw Analytical Total
- •23.4.2 The Shape of the EDS Spectrum
- •23.5 Best Practices for Analysis of Rough Bulk Samples
- •23.6 Particle Analysis
- •Particle Sample Preparation: Bulk Substrate
- •The Importance of Beam Placement
- •Overscanning
- •“Particle Mass Effect”
- •“Particle Absorption Effect”
- •The Analytical Total Reveals the Impact of Particle Effects
- •Does Overscanning Help?
- •23.6.6 Peak-to-Background (P/B) Method
- •Specimen Geometry Severely Affects the k-ratio, but Not the P/B
- •Using the P/B Correspondence
- •23.7 Summary
- •References
- •24: Compositional Mapping
- •24.2 X-Ray Spectrum Imaging
- •24.2.1 Utilizing XSI Datacubes
- •24.2.2 Derived Spectra
- •SUM Spectrum
- •MAXIMUM PIXEL Spectrum
- •24.3 Quantitative Compositional Mapping
- •24.4 Strategy for XSI Elemental Mapping Data Collection
- •24.4.1 Choosing the EDS Dead-Time
- •24.4.2 Choosing the Pixel Density
- •24.4.3 Choosing the Pixel Dwell Time
- •“Flash Mapping”
- •High Count Mapping
- •References
- •25.1 Gas Scattering Effects in the VPSEM
- •25.1.1 Why Doesn’t the EDS Collimator Exclude the Remote Skirt X-Rays?
- •25.2 What Can Be Done To Minimize gas Scattering in VPSEM?
- •25.2.2 Favorable Sample Characteristics
- •Particle Analysis
- •25.2.3 Unfavorable Sample Characteristics
- •References
- •26.1 Instrumentation
- •26.1.2 EDS Detector
- •26.1.3 Probe Current Measurement Device
- •Direct Measurement: Using a Faraday Cup and Picoammeter
- •A Faraday Cup
- •Electrically Isolated Stage
- •Indirect Measurement: Using a Calibration Spectrum
- •26.1.4 Conductive Coating
- •26.2 Sample Preparation
- •26.2.1 Standard Materials
- •26.2.2 Peak Reference Materials
- •26.3 Initial Set-Up
- •26.3.1 Calibrating the EDS Detector
- •Selecting a Pulse Process Time Constant
- •Energy Calibration
- •Quality Control
- •Sample Orientation
- •Detector Position
- •Probe Current
- •26.4 Collecting Data
- •26.4.1 Exploratory Spectrum
- •26.4.2 Experiment Optimization
- •26.4.3 Selecting Standards
- •26.4.4 Reference Spectra
- •26.4.5 Collecting Standards
- •26.4.6 Collecting Peak-Fitting References
- •26.5 Data Analysis
- •26.5.2 Quantification
- •26.6 Quality Check
- •Reference
- •27.2 Case Study: Aluminum Wire Failures in Residential Wiring
- •References
- •28: Cathodoluminescence
- •28.1 Origin
- •28.2 Measuring Cathodoluminescence
- •28.3 Applications of CL
- •28.3.1 Geology
- •Carbonado Diamond
- •Ancient Impact Zircons
- •28.3.2 Materials Science
- •Semiconductors
- •Lead-Acid Battery Plate Reactions
- •28.3.3 Organic Compounds
- •References
- •29.1.1 Single Crystals
- •29.1.2 Polycrystalline Materials
- •29.1.3 Conditions for Detecting Electron Channeling Contrast
- •Specimen Preparation
- •Instrument Conditions
- •29.2.1 Origin of EBSD Patterns
- •29.2.2 Cameras for EBSD Pattern Detection
- •29.2.3 EBSD Spatial Resolution
- •29.2.5 Steps in Typical EBSD Measurements
- •Sample Preparation for EBSD
- •Align Sample in the SEM
- •Check for EBSD Patterns
- •Adjust SEM and Select EBSD Map Parameters
- •Run the Automated Map
- •29.2.6 Display of the Acquired Data
- •29.2.7 Other Map Components
- •29.2.10 Application Example
- •Application of EBSD To Understand Meteorite Formation
- •29.2.11 Summary
- •Specimen Considerations
- •EBSD Detector
- •Selection of Candidate Crystallographic Phases
- •Microscope Operating Conditions and Pattern Optimization
- •Selection of EBSD Acquisition Parameters
- •Collect the Orientation Map
- •References
- •30.1 Introduction
- •30.2 Ion–Solid Interactions
- •30.3 Focused Ion Beam Systems
- •30.5 Preparation of Samples for SEM
- •30.5.1 Cross-Section Preparation
- •30.5.2 FIB Sample Preparation for 3D Techniques and Imaging
- •30.6 Summary
- •References
- •31: Ion Beam Microscopy
- •31.1 What Is So Useful About Ions?
- •31.2 Generating Ion Beams
- •31.3 Signal Generation in the HIM
- •31.5 Patterning with Ion Beams
- •31.7 Chemical Microanalysis with Ion Beams
- •References
- •Appendix
- •A Database of Electron–Solid Interactions
- •A Database of Electron–Solid Interactions
- •Introduction
- •Backscattered Electrons
- •Secondary Yields
- •Stopping Powers
- •X-ray Ionization Cross Sections
- •Conclusions
- •References
- •Index
- •Reference List
- •Index
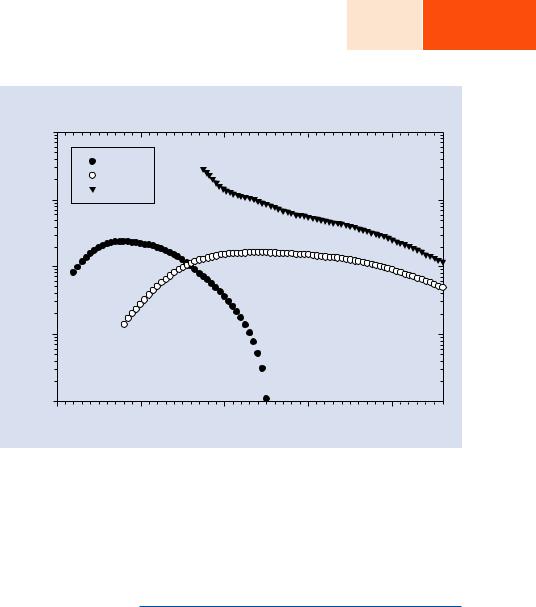
47 |
4 |
4.3 · X-Ray Continuum (bremsstrahlung)
. Fig. 4.8 Product of the ionization cross section, the fluorescence yield, and the relative weights of lines for the most intense member of the K-, L-, and M-shells for E0 = 30 keV
Product of ionization cross section, relative transition probability and fluorescence yield
|
1 |
|
|
|
|
|
|
K-shell |
|
|
|
|
|
L-shell |
|
|
|
|
|
M-shell |
|
|
|
|
0.1 |
|
|
|
|
) |
|
|
|
|
|
2 |
|
|
|
|
|
cm |
|
|
|
|
|
-20 |
0.01 |
|
|
|
|
QxFxw (x10 |
|
|
|
|
|
|
|
|
|
|
|
|
0.001 |
|
|
|
|
0.0001 |
|
|
|
|
|
|
0 |
20 |
40 |
60 |
80 |
Atomic number (Z)
strong differences in the relative abundance of the X-rays produced by different elements. This plot also reveals that over certain atomic number ranges, two different atomic shells can be excited for each element, for example, K and L for Z = 16 to Z = 50, and L and M for Z = 36 to Z = 92. For lower values of E0, these atomic number ranges will be diminished.
X-Ray Intensity Emitted from Thick, Solid Specimens
A thick specimen is one with sufficient thickness so that it contains the full electron interaction volume, which generally requires a thickness of at least a few micrometers for most choices of composition and incident beam energy. Within the interaction volume, the complete range of elastic and inelastic scattering events occur. X-ray generation for each atom species takes place across the full energy range of the ionization cross section from the initial value corresponding to the energy of the incident beam as it enters the specimen down to the ionization energy of each atom species. Based upon experimental measurements, the X-ray intensity emitted from thick targets is found to follow an expression of the form
p ( |
|
0 |
|
c ) |
0 |
|
p [ |
] |
\ |
|
I ≈ i |
E |
|
− E |
|
/ E |
n ≈ i U −1 n |
|
(4.8) |
where ip is the beam current, and n is a constant depending on the particular element and shell (Lifshin et al. 1980). The value of n is typically in the range 1.5–2.0. Equation 4.8 is plotted for an exponent of n = 1.7 in . Fig. 4.9. The intensity
rises rapidly from a zero value at U = 1. For a reasonably efficient degree of X-ray excitation, it is desirable to select E0 so that U0 > 2 for the highest value of Ec among the elements of interest.
4.3\ X-Ray Continuum (bremsstrahlung)
Simultaneously with the inner shell ionization events that lead to characteristic X-ray emission, a second physical process operates to generate X-rays, the “braking radiation,” or bremsstrahlung, process. As illustrated in . Fig. 4.10, because of the repulsion that the beam electron experiences in the negative charge cloud of the atomic electrons, it undergoes deceleration and loses kinetic energy, which is released as a photon of electromagnetic radiation. The energy lost due to deceleration can take on any value from a slight deceleration involving the loss of a few electron volts up to the loss of the total kinetic energy carried by the beam electron in a single event. Thus, the bremsstrahlung X-rays span all energies from a practical threshold of 100 eV up to the incident beam energy, E0, which corresponds to an incident beam electron suffering total energy loss by deceleration in the Coulombic field of a surface atom as the beam electron enters the target and before it has lost any energy in any other inelastic scattering events. The braking radiation process thus forms a continuous energy spectrum, also referred to as the “X-ray continuum,” from 100 eV to E0, which is the so-called Duane–Hunt limit. The X-ray continuum forms a background beneath any characteristic X-rays produced by the atoms. The bremsstrahlung process is anisotropic, being somewhat peaked in the
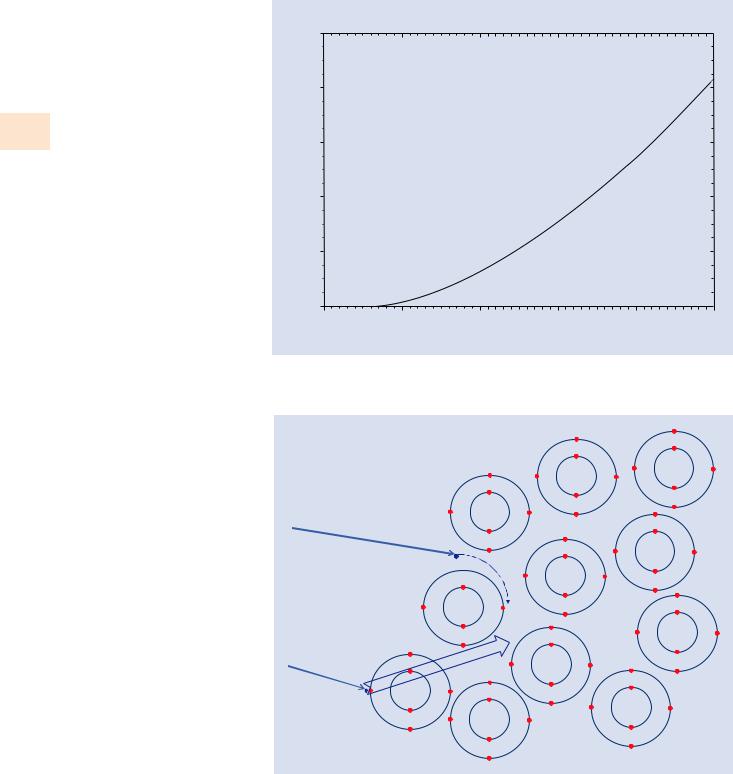
4
48\ |
Chapter 4 · X-Rays |
|
|
|
|
|
|
|
. Fig. 4.9 Characteristic X-ray inten- |
|
|
|
|
|
|
|
|
sity emitted from a thick specimen; |
|
|
|
Relative X-ray intensity (thick specimen) |
|
|
||
exponent n = 1.7 |
|
50 |
|
|
|
|
|
|
|
|
intensityray-X |
40 |
|
|
|
|
|
|
|
30 |
|
|
|
|
|
|
|
|
|
|
|
|
|
|
|
|
|
Relative |
20 |
|
|
|
|
|
|
|
|
|
|
|
|
|
|
|
|
|
10 |
|
|
|
|
|
|
|
|
0 |
|
|
|
|
|
|
|
|
0 |
2 |
4 |
6 |
8 |
10 |
Overvoltage, U = E0/Ec
. Fig. 4.10 Schematic illustration of the braking radiation (bremsstrahlung) process giving rise to the X-ray continuum
Eν
Eν =E0
direction of the electron travel. In thin specimens where the beam electron trajectories are nearly aligned, this anisotropy can result in a different continuum intensity in the forward direction along the beam relative to the backward direction.
However, in thick specimens, the near-randomization of the beam electron trajectory segments by elastic scattering effectively smooths out this anisotropy, so that the X-ray continuum is effectively rendered isotropic.

4.3 · X-Ray Continuum (bremsstrahlung)
4.3.1\ X-Ray Continuum Intensity
The intensity of the X-ray continuum, Icm , at an energy Eν is described by Kramers (1923) as
I |
|
≈ i Z |
E |
− E |
/ E |
\ |
(4.9) |
|
cm |
p ( |
0 |
ν ) |
ν |
|
where ip is the incident beam current and Z is the atomic number. For a particular value of the incident energy, E0, the intensity of the continuum decreases rapidly relative to lower photon energies as Eν approaches E0, the Duane–Hunt limit.
An important parameter in electron-excited X-ray microanalysis is the ratio of the characteristic X-ray intensity to the X-ray continuum intensity at the same energy, Ech = Eν, often referred to as the “peak-to-background, P/B.” The P/B can be estimated from Eqs. (4.8) and (4.9) with the approximation that Eν ≈ Ec so that Eq. (4.9) can be rewritten as—
I |
|
≈ i Z |
E |
|
− E |
c ) |
/ E |
≈ i Z U −1 |
\ |
(4.10) |
|
|
cm |
p ( |
|
0 |
|
c |
p ( |
) |
|
Taking the ratio of Eqs. (4.8) and (4.10) gives
( |
)( |
) |
n −1 |
|
|
P / B ≈ 1 / Z |
U −1 |
\ |
(4.11) |
||
|
|
|
|
|
The P/B is plotted in . Fig. 4.11 with the assumption that n = 1.7, where it is seen that at low overvoltages, which are often used in electron-excited X-ray microanalysis, the characteristic intensity is low relative to higher values of U, and the intensity rises rapidly with U, while the P/B increases rapidly at low overvoltage but then more slowly as the overvoltage increases.
49 |
|
4 |
|
|
|
4.3.2\ The Electron-Excited X-Ray Spectrum,
As-Generated
The electron-excited X-ray spectrum generated within the target thus consists of characteristic and continuum X-rays and is shown for pure carbon with E0 =20 keV in . Fig. 4.12, as calculated with the spectrum simulator in NIST Desktop Spectrum Analyzer (Fiori et al. 1992), using the Pouchou and Pichoir expression for the K-shell ionization cross section and the Kramers expression for the continuum intensity (Pouchou and Pichoir 1991; Kramers 1923). Because of the energy dependence of the continuum given by Eq. 4.10, the generated X-ray continuum has its highest intensity at the lowest photon energy and decreases at higher photon energies, reaching zero intensity at E0. By comparison, the energy span of the characteristic C–K peak is its natural width of only 1.6 eV, which is related to the lifetime of the excited K-shell vacancy. The energy width for K-shell emission up to 25 keV photon energy is shown in . Fig. 4.2 (Krause and Oliver 1979). For photon energies below 25 keV, the characteristic X-ray peaks from the K-, L-, and M- shells have natural widths less than 10 eV. In the calculated spectrum of . Fig. 4.12, the C–K peak is therefore plotted as a narrow line. (X-ray peaks are often referred to as “lines” in the literature, a result of their appearance in highenergy resolution measurements of X-ray spectra by diffrac- tion-based X-ray spectrometers.) The X-ray spectra as-generated in the target for carbon, copper, and gold are compared in . Fig. 4.13, where it can be seen that at all photon energies the intensity of the X-ray continuum increases with Z, as given by Eq. 4.9. The increased complexity of the characteristic X-rays at higher Z is also readily apparent.
. Fig. 4.11 X-ray intensity emitted from a thick specimen and P/B, both as a function of overvoltage with exponent n = 1.7
8
6
and P/B |
|
intensityRelative |
4 |
|
2
0
1.0
Relative X-ray intensity and P/B (thick specimen)
P/B
Relative X-ray intensity
1.5 |
2.0 |
2.5 |
3.0 |
3.5 |
4.0 |
|
|
Overvoltage, U = E0/Ec |
|
|
|

50\ Chapter 4 · X-Rays
. Fig. 4.12 Spectrum of pure carbon as-generated within the target calculated for E0 = 20 keV with the spectrum
simulator in Desktop Spectrum Ana- 1e+7 lyzer
1e+6
1e+5
4
. Fig. 4.13 Spectra of pure carbon, copper, and gold as-generated within the target calculated for E0 = 20 keV with the spectrum simulator in Desktop Spectrum Analyzer (Fiori et al. 1992)
Intensity |
1e+4 |
|
1e+3 |
||
|
1e+2
1e+1
1e+0
0
|
1e+8 |
|
|
1e+7 |
|
|
1e+6 |
|
Intensity |
1e+5 |
|
1e+4 |
||
|
||
|
1e+3 |
1e+2
1e+1
1e+0
0
As-generated electron-excited X-ray spectrum of carbon
5 |
10 |
15 |
20 |
|
X-ray photon energy (keV) |
|
|
As-generated electron-excited X-ray spectra
C
Cu
Au
5 |
10 |
15 |
20 |
|
X-ray photon energy (keV) |
|
|
4.3.3\ Range of X-ray Production
As the beam electrons scatter inelastically within the target and lose energy, inner shell ionization events can be produced from U0 down to U = 1, so that depending on E0 and the value(s) of Ec represented by the various elements in the target, X-rays will be generated over a substantial portion of the interaction volume. The “X-ray range,” a
crude estimate of the limiting range of X-ray generation, can be obtained from a simple modification of the Kanaya–Okayama range equation (IV-5) to compensate for the portion of the electron range beyond which the energy of beam electrons has deceased below a specific value of Ec:
K−O ( |
|
) |
|
( |
|
) 0 |
c |
|
|
|
|
R |
nm |
|
= 27.6 |
|
A / Z0.89 ρ |
E 1.67 |
− E 1.67 |
|
\ |
\ |
(4.12) |
|
|
|
|
|
|
|
|
|
|
|

4.3 · X-Ray Continuum (bremsstrahlung)
. Table 4.2 lists calculations of the range of generation for copper K-shell X-rays (Ec = 8.98 keV) produced in various host elements, for example, a situation in which copper is present at a low level so it has a negligible effect on the overall Kanaya–Okayama range. As the incident beam energy decreases to E0 = 10 keV, the range of production of copper K-shell X-rays decreases to a few hundred nanometers because of the very low overvoltage, U0 = 1.11. The X-ray range
. Table 4.2 Range of Cu K-shell (Ec = 8.98 keV) X-ray
generation in various matrices
Matrix |
25 keV |
|
|
20 keV |
|
|
15 keV |
10 keV |
|
|
||||||
|
|
|
|
|
|
|
|
|
|
|
|
|
|
|
||
C |
|
|
|
|
6.3 μm |
|
|
3.9 μm |
|
|
1.9 μm |
|
270 nm |
|
|
|
|
|
|
|
|
|
|
|
|
|
|
|
|||||
Si |
5.7 μm |
|
|
3.5 μm |
|
|
1.7 μm |
250 nm |
|
|
||||||
Fe |
1.9 μm |
|
|
1.2 μm |
|
|
570 nm |
83 nm |
|
|
||||||
Au |
1.0 μm |
|
|
630 nm |
|
|
310 nm |
44 nm |
|
|
||||||
a |
10 |
|
|
|
|
|
Range of X-ray production in carbon |
|
|
|
||||||
|
|
|
|
|
|
|
|
|
|
|
|
|
|
|
||
|
|
|
|
|
|
|
|
|
|
|
|
|
|
|||
|
8 |
|
|
|
|
|
|
|
|
|
|
|
|
|
|
|
|
|
|
|
|
|
|
|
Electron range |
|
|
|
|
|
|
||
|
|
|
|
|
|
|
|
|
|
|
|
|||||
(micrometers) |
|
|
|
|
|
|
|
|
C K X-rays |
|
|
|
|
|
|
|
|
|
|
|
|
|
|
|
AIK X-rays |
|
|
|
|
|
|
|
|
6 |
|
|
|
|
|
|
|
TiK X-rays |
|
|
|
|
|
|
|
|
|
|
|
|
|
|
|
|
|
|
|
|
|
|
|
||
|
|
|
|
|
|
|
|
FeK X-rays |
|
|
|
|
|
|
|
|
|
|
|
|
|
|
|
|
|
CuK X-rays |
|
|
|
|
|
|
|
Range |
4 |
|
|
|
|
|
|
|
|
|
|
|
|
|
|
|
|
|
|
|
|
|
|
|
|
|
|
|
|
|
|
||
|
|
|
|
|
|
|
|
|
|
|
|
|
|
|
|
|
|
2 |
|
|
|
|
|
|
|
|
|
|
|
|
|
|
|
|
0 |
|
|
|
|
|
|
|
|
|
|
|
|
|
|
|
|
|
|
|
|
|
|
|
|
|
|
|
|
|
|
|
|
|
0 |
|
5 |
10 |
15 |
20 |
|
25 |
30 |
|||||||
|
|
|
|
|
|
|
|
|
Beam energy (keV) |
|
|
|
||||
c |
3.0 |
|
|
|
|
|
Range of X-ray production in copper |
|
|
|
||||||
|
|
|
|
|
|
|
|
|
|
|
|
|
|
|
||
|
|
|
|
|
|
|
|
|
|
|
|
|
|
|
||
|
2.5 |
|
|
|
|
|
|
|
Electron range |
|
|
|
|
|
||
|
|
|
|
|
|
|
|
|
|
|
|
|
||||
|
|
|
|
|
|
|
|
|
C K X-rays |
|
|
|
|
|
|
|
(micrometers) |
|
|
|
|
|
|
|
|
AIK X-rays |
|
|
|
|
|
|
|
2.0 |
|
|
|
|
|
|
|
TiK X-rays |
|
|
|
|
|
|
|
|
|
|
|
|
|
|
|
|
|
FeK X-rays |
|
|
|
|
|
|
|
|
|
|
|
|
|
|
|
|
CuK X-rays |
|
|
|
|
|
|
|
Range |
1.5 |
|
|
|
|
|
|
|
|
|
|
|
|
|
|
|
|
|
|
|
|
|
|
|
|
|
|
|
|
|
|
||
1.0 |
|
|
|
|
|
|
|
|
|
|
|
|
|
|
|
|
|
|
|
|
|
|
|
|
|
|
|
|
|
|
|
|
|
|
0.5 |
|
|
|
|
|
|
|
|
|
|
|
|
|
|
|
|
0.0 |
|
|
|
|
|
|
|
|
|
|
|
|
|
|
|
|
0 |
5 |
10 |
15 |
20 |
|
25 |
30 |
|
Beam energy (keV)
51 |
|
4 |
|
|
|
in various matrices for the generation of various characteristic X-rays spanning a wide range of Ec is shown in . Fig. 4.14a–d.
4.3.4\ Monte Carlo Simulation of X-Ray
Generation
The X-ray range given by Eq. 4.12 provides a single value that captures the limit of the X-ray production but gives no information on the complex distribution of X-ray production within the interaction volume. Monte Carlo electron simulation can provide that level of detail (e.g., Drouin et al., 2007; Joy, 2006; Ritchie, 2015), as shown in . Fig. 4.15a, where the electron trajectories and the associated emitted photons of Cu K-L3 are shown superimposed. For example, the limit of production of Cu K-L3 that occurs when energy loss causes the beam electron energy to fall below the Cu K-shell excitation energy (8.98 keV) can be seen in the electron trajectories (green) that extend beyond the region of X-ray production (red). The effects of the host element on the
b 10 |
|
|
|
|
|
Range of X-ray production in aluminum |
|
|
|
||||||||
|
|
|
|
|
|
|
|
|
|
|
|
|
|
|
|||
|
8 |
|
|
|
|
|
|
|
|
|
|
|
|
|
|
|
|
|
|
|
|
|
|
|
|
|
Electron range |
|
|
|
|
|
|
||
|
|
|
|
|
|
|
|
|
|
|
|
||||||
(micrometers) |
|
|
|
|
|
|
|
|
|
C K X-rays |
|
|
|
|
|
|
|
|
|
|
|
|
|
|
|
|
AIK X-rays |
|
|
|
|
|
|
|
|
|
6 |
|
|
|
|
|
|
|
|
TiK X-rays |
|
|
|
|
|
|
|
|
|
|
|
|
|
|
|
|
FeK X-rays |
|
|
|
|
|
|
|
|
|
|
|
|
|
|
|
|
|
|
CuK X-rays |
|
|
|
|
|
|
|
Range |
4 |
|
|
|
|
|
|
|
|
|
|
|
|
|
|
|
|
|
|
|
|
|
|
|
|
|
|
|
|
|
|
|
|
||
|
|
|
|
|
|
|
|
|
|
|
|
|
|
|
|
|
|
|
2 |
|
|
|
|
|
|
|
|
|
|
|
|
|
|
|
|
|
0 |
|
|
|
|
|
|
|
|
|
|
|
|
|
|
|
|
|
|
|
|
|
|
|
|
|
|
|
|
|
|
|
|
|
|
|
0 |
|
|
|
5 |
10 |
15 |
|
20 |
25 |
30 |
||||||
|
|
|
|
|
|
|
|
|
|
Beam energy (keV) |
|
|
|
|
|||
d 2.0 |
|
|
|
|
Range of X-ray production in gold |
|
|
|
|||||||||
|
|
|
|
|
|
|
|
|
|
|
|
|
|||||
|
|
|
|
|
|
|
|
|
|
|
|
|
|
||||
|
|
|
|
|
|
|
|
|
|
|
|
|
|
|
|
||
|
|
|
|
|
|
|
|
|
|
Electron range |
|
|
|
|
|
||
|
|
|
|
|
|
|
|
|
|
|
|
|
|
||||
(micrometers)Range |
1.5 |
|
|
|
|
|
|
|
C K X-rays |
|
|
|
|
|
|
|
|
|
|
|
|
|
|
|
|
|
AIK X-rays |
|
|
|
|
|
|
|
|
|
|
|
|
|
|
|
|
|
|
TiK X-rays |
|
|
|
|
|
|
|
|
|
|
|
|
|
|
|
|
|
FeK X-rays |
|
|
|
|
|
|
|
|
1.0 |
|
|
|
|
|
|
|
CuK X-rays |
|
|
|
|
|
|
|
|
|
|
|
|
|
|
|
|
|
|
|
|
|
|
|
|
||
|
0.5 |
|
|
|
|
|
|
|
|
|
|
|
|
|
|
|
|
|
0.0 |
|
|
|
|
|
|
|
|
|
|
|
|
|
|
|
|
|
0 |
|
5 |
10 |
15 |
|
20 |
25 |
30 |
||||||||
|
|
|
|
|
|
|
|
|
|
Beam energy (keV) |
|
|
|
|
. Fig. 4.14 a X-ray range as a function of E0 for generation of K-shell X-rays of C, Al, Ti, Fe, and Cu in a C matrix. b X-ray range as a function of E0 for generation of K-shell X-rays of C, Al, Ti, Fe, and Cu in an Al matrix.
c X-ray range as a function of E0 for generation of K-shell X-rays of C, Al, Ti, Fe, and Cu in a Cu matrix. d X-ray range as a function of E0 for generation of K-shell X-rays of C, Al, Ti, Fe, and Cu in an Au matrix
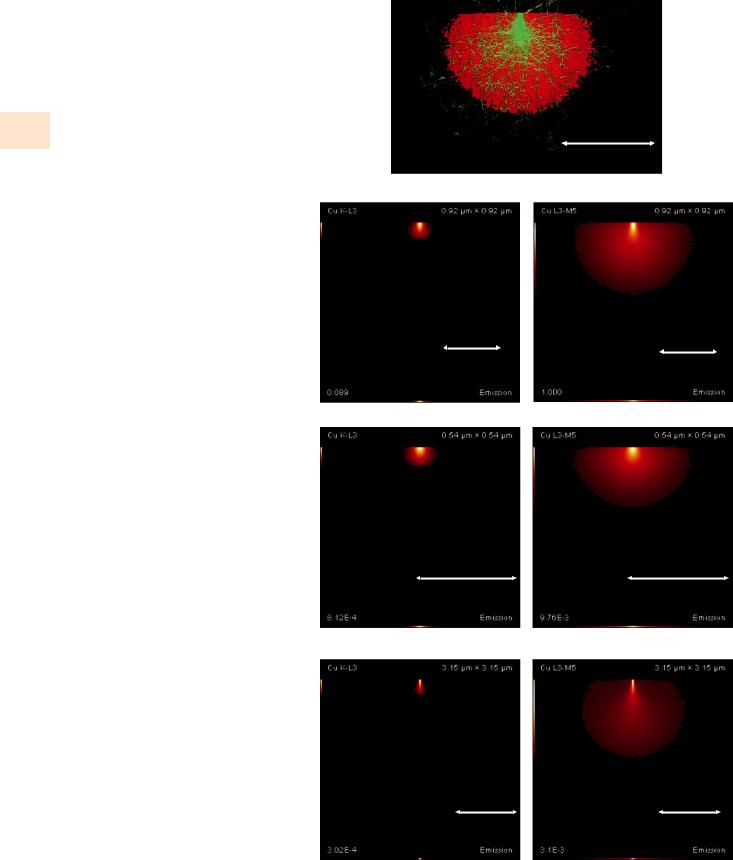
52\ Chapter 4 · X-Rays
. Fig. 4.15 a Monte Carlo simulation (DTSA-II) of electron trajectories and associated Cu K-shell X-ray generation in pure copper; E0 = 20 keV.
b Monte Carlo simulation (DTSA-II) of the distribution of Cu K-shell and L-shell X-rays in a Cu matrix with E0 = 10 keV showing the X-rays that escape. c Monte Carlo simulation (DTSA-II) of the distribution of Cu K-shell and L-shell X-rays that escape in Au-1 % Cu with E0 = 10 keV. d Monte Carlo simula-
4 tion (DTSA-II) of the distribution of Cu K-shell and L-shell X-rays that escape in C-1 % Cu with E0 = 10 keV (Ritchie 2015)
a
|
|
Cu |
|
|
|
|
|
|
|
|
Cu K-L3 |
|
|
1 µm |
|||
|
|
E0 = 20 keV |
|
|
|
|
|
|
b |
Monte Carlo (DTSA-II) simulation of Cu X-ray production in Cu; E0 = 10 keV |
|||||||
|
|
|
|
|
|
|
|
|
|
Cu K-L3 |
0.92 mm x 0.92 mm |
Cu L3-M5 |
092 mm x 0.92 mm |
|
|
|
|
Cu K-L3 in Cu |
|
|
|
|
|
Cu L3-M5 in Cu |
|||
|
|
|
|
E0 = 10 keV |
|
|
|
|
|
||||
|
|
|
|
|
|
|
|
|
|
|
E0 = 10 keV |
||
|
|
|
|
250 nm |
|
|
|
|
|
250 nm |
|||
|
|
|
|
|
|
|
|
|
|
|
|||
|
|
|
|
|
|
|
|
|
|
|
|||
|
|
|
|
|
|
|
1.000 |
|
|
|
Emission |
||
0.089 |
|
|
Emission |
|
|||||||||
c |
Monte Carlo (DTSA-II) simulation of Cu X-ray production in Au; E0 = 10 keV |
||||||||||||
|
|
|
|
|
|
|
|
|
|
|
|
|
|
|
Cu K-L3 |
0.54 mm x 0.54 mm |
|
Cu L3-M5 |
0.54 mm x 0.54 mm |
|
|
|
|
Cu K-L in Au |
|
|
|
|
Cu L3-M5 in Au |
||||
|
|
|
3 |
|
|
|
|
|
E0 = 10 keV |
||||
|
|
|
|
E0 = 10 keV |
|
|
|
|
|||||
|
|
|
|
250 nm |
|
|
|
|
|
250 nm |
|||
|
|
|
|
|
|
|
|
|
|
|
|
|
|
8.12E-4 |
|
Emission |
|
9.76E-3 |
|
Emission |
|||||||
d |
Monte Carlo (DTSA-II) simulation of Cu X-ray production in C; E0 = 10 keV |
||||||||||||
|
|
|
|
|
|
|
|
|
|
|
|
|
|
|
Cu K-L3 |
3.15 mm x 3.15 mm |
|
Cu L3-M5 |
3.15 mm x 3.15 mm |
|
Cu K-L3 in C |
|
Cu L3-M5 in C |
|||
|
E0 = 10 keV |
|
E0 = 10 keV |
|||
|
1 µm |
|
1 µm |
|||
|
|
|
|
|
|
|
3.02E-4 |
Emission |
3.1E-3 |
Emission |
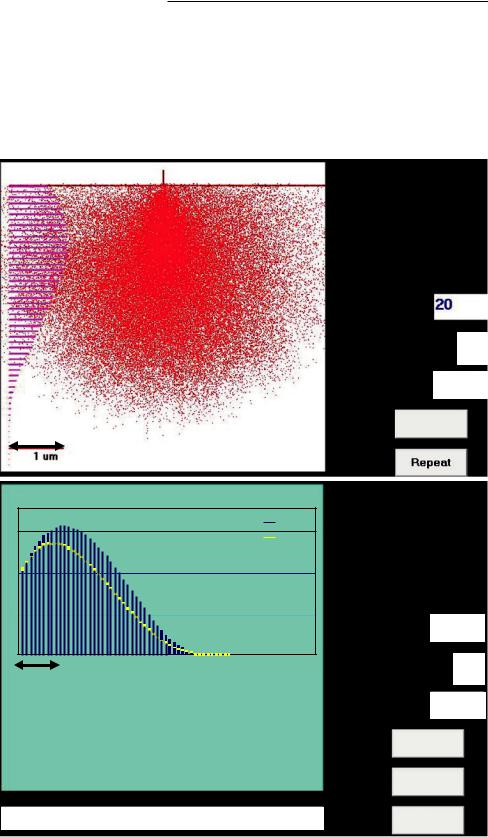
4.3 · X-Ray Continuum (bremsstrahlung)
emission volumes for Cu K-shell and L-shell X-ray generation in three different matrices—C, Cu, and Au—is shown in
. Fig. 4.15a–c using DTSA-II (Ritchie 2015). The individual maps of X-ray production show the intense zone of X-ray generation starting at and continuing below the beam impact point and the extended region of gradually diminishing X-ray generation. In all three matrices, there is a large difference in the generation volume for the Cu K-shell and Cu L-shell X-rays as a result of the large difference in overvoltage at E0 = 10 keV: CuK U0 = 1.11 and CuL U0 = 10.8.
53 |
|
4 |
|
|
|
4.3.5\ X-ray Depth Distribution Function,
ϕ(ρz)
The distribution of characteristic X-ray production as a function of depth, designated “ϕ(ρz)” in the literature of quantitative electron-excited X-ray microanalysis, is a critical parameter that forms the basis for calculating the compositionally dependent correction (“A” factor) for the loss of X-rays due to photoelectric absorption. As shown in . Fig. 4.16 for Si with E0 =20 keV, Monte Carlo electron trajectory simulation provides a
. Fig. 4.16 a Monte Carlo calculation of the interaction volume and X-ray production in Si with E0 = 20 keV. The histogram construction of the X-ray depth distribution ϕ(ρz) is illustrated. (Joy Monte Carlo simulation). b ϕ(ρz) distribution of generated Si K-L3 X-rays in Si with E0 = 20 keV, and the effect of absorption from each layer, giving the fraction,
f(χ)depth, that escapes from each layer. The cumulative escape from
all layers is f(χ) = 0.80. (Joy Monte Carlo simulation) (Joy 2006)
a
Energy (keV) |
20 |
|
|
|
|
Tilt/TOA 0
Number 5000
Select
Si
1 µm |
|
Repeat |
|
|
|
b
Phiroz
f(chi)
|
Energy (keV) |
20 |
|
|
|
|
|
||
|
Tilt/TOA |
|
|
0 |
|
|
|
|
|
1 µm |
|
|
|
|
|
Number |
|
20000 |
|
|
|
|
|
|
|
|
Select |
|
|
Si |
Repeat |
|
||
|
|
X-ray volume = 3.76312um^3 – f(chi) = 0.8 |
Exit |
|